- The β-lactam class of antibiotics includes penicillins, cephalosporins, carbapenems, and monobactams; many, particularly the newer examples, exhibit a broad spectrum of activity against both Gram negative and Gram positive bacteria.
- These agents inhibit the transpeptidase enzyme, also called penicillin-binding protein (PBP), which crosslinks peptidoglycan strands to provide strength and rigidity to the bacterial cell wall.
- Widespread resistance to β-lactam antibiotics has limited their clinical use, although they remain of value for the treatment of susceptible bacterial infections.
There are many β-lactam antibiotics used clinically; these include the penicillins (usually as their sodium salts) (Table 5.1.1), the cephalosporins (Table 5.1.2), the carbapenems (Table 5.1.3), and aztreonam, a monobactam (Table 5.1.4). The structures of the β-lactam antibiotics are shown in Figures 5.1.1–5.1.4 and, as the name implies, all contain the β-lactam1 ring functionality (highlighted in red), which is key to their antibacterial activity.
Table 5.1.1 Therapeutic indications for the penicillin antibiotics.
Penicillin antibiotic | R1 (R2 = Na unless stated otherwise) | Indications |
Benzylpenicillin | PhCH2 | Bacterial meningitis, endocarditis, cellulitis |
Benzathine penicillin | PhCH2 | Prevention of rheumatic fever |
(Benzathine benzylpenicillin) | (R2 = (PhCH2NHCH2)2) | Infections susceptible to prolonged low concentrations of benzylpenicillin, e.g. latent syphilis |
Procaine penicillin | PhCH2 | Respiratory tract infections (RTIs), syphilis, cellulitis, erysipelas |
(Procaine benzylpenicillin) | (R2 = 4-H2NC6H4CO2CH2CH2NEt2) | Intramuscular injections |
Phenoxymethylpenicillin | PhOCH2 | Cellulitis, throat infections |
Ampicillin | ![]() | Urinary tract infection (UTI), endocarditis |
Amoxicillin | ![]() | Otitis media, endocarditis, community-acquired pneumonia (CAP), UTIs, Lyme disease, H. pylori eradication, sinusitis, otitis media |
Amoxicillin with clavulanic acid | ![]() | Hospital-acquired pneumonia (HAP) |
Dicloxacillin | ![]() | Uncomplicated skin and soft-tissue infections (SSTIs), osteomyelitis, endocarditis |
Flucloxacillin | ![]() | Uncomplicated SSTIs, osteomyelitis, endocarditis |
Temocillin | See Figure 5.1.1 | UTIs |
Piperacillin with tazobactam | ![]() | HAP, community-acquired septicaemia, hospital-acquired septicaemia |
Pivmecillinam | See Figure 5.1.1 | UTIs |
Ticarcillin with clavulanic acid | See Figure 5.1.1 | HAP, community-acquired septicaemia, hospital-acquired septicaemia |
Table 5.1.2 Therapeutic indications for the cephalosporin antibiotics (colours represent generations: first, second, third, and fourth).
Table 5.1.3 Therapeutic indications for the carbapenem antibiotics.
Table 5.1.4 Therapeutic indications for the monobactam aztreonam.
Monobactam | Indications |
Aztreonam | Chronic pulmonary P. aeruginosa infection in patients with cystic fibrosis (delivered intravenously or by inhalation), UTIs, gonorrhoea |
Figure 5.1.1 General structure of the penicillin antibiotics and some β-lactamase inhibitors. The β-lactam ring (shown in red in the penicillin general structure) and all other substituents within the box, with the absolute stereochemistry indicated, are essential for antibacterial activity
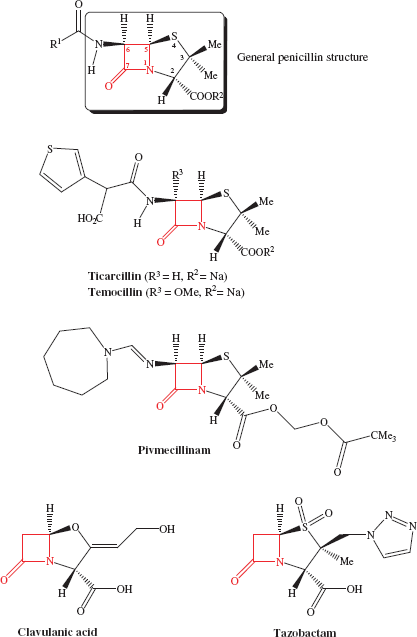
5.1.1 Discovery
The discovery of penicillin represents a pivotal moment in medical history and the sequence of events which led to it illustrates the serendipity sometimes associated with the isolation and subsequent development of a therapeutic agent.
Alexander Fleming was the Medical Director of the Inoculation Department of St Mary’s Hospital in London in 1928 and was interested in the cultivation of bacteria, in particular Haemophilus influenzae. As you are probably already aware, several factors conspired to result in Fleming’s discovery of the antibacterial activity of penicillin:
- Moulds of the relatively rare Penicillium notatum were being cultivated (for the development of vaccines) in the department on the floor below Fleming’s laboratory and this probably resulted in the contamination of the laboratory air, and so the Petri dishes in Fleming’s laboratory, by these spores.
- The contaminated Petri dishes were not incubated, which would normally promote the growth of bacteria and not fungi, and this allowed the Penicillium mould to grow preferentially.
- These events occurred during a particularly cool July, which also allowed the preferential growth of the Penicillium mould. A subsequent rise in climatic temperature at the end of the UK summer facilitated the re-establishment of Staphylococcus colonies, but the penicillin mould (not normally developed on such plates) was sufficiently large to demonstrate a zone of staphylococcal growth inhibition (Hare, 1970).
This sequence of events resulted in the growth of Staphylococcus aureus being inhibited by Penicillium notatum, the mould that produced significant amounts of penicillin. Further studies then demonstrated that penicillin was useful in the eradication of Haemophilus influenzae strains, particularly those derived from throat swabs (Fleming, 1929).
Initial attempts to isolate penicillin were performed by Raistrick and Clutterbuck, in the School of Hygiene and Tropical Medicine in London. An ether extract of the mould lacked any antibacterial activity, but Holt deduced that penicillin could be extracted into organic solvents from slightly acidic solutions, suggesting that penicillin was probably a weak acid.
In 1938, Ernst Chain and Howard Florey and their colleagues investigated the effects of penicillin on bacteria, showing that it caused cell-wall lysis. The fact that penicillin could be extracted into organic solvents demonstrated that penicillin was a small molecule and probably not an enzyme. Subsequent funding by the Rockefeller Foundation ensured that penicillin could be produced in sufficient quantities for further scientific investigation, allowing Chain to isolate approximately 100 mg of penicillin in 1940.
With modest amounts of sufficiently pure penicillin available, study of its antibiotic effects against streptococci, staphylococci, and clostridium bacteria in infected mice demonstrated its efficacy: mice injected with several small doses of penicillin survived, while untreated mice and those given a single dose did not (Chain et al., 1940). Tests in human volunteers rapidly followed, confirming that oral administration destroyed the pencillin structure and antibiotic effect, while injection or infusion was effective in achieving detectable amounts of active drug systemically. In 1941, at a hospital in Oxford, England, the first patient with a serious infection, due to both staphylococci and streptococci, was recruited to the trial and the efficacy of penicillin in man was demonstrated; at this time, despite the attempts to increase the quantity of penicillin for treatment by using bedpans as larger culture vessels, production struggled to provide sufficient amounts, so renally excreted unchanged penicillin was recovered from the urine for re-use (Fletcher, 1984). Fleming, Chain, and Florey shared the Nobel Prize for Physiology or Medicine in 1945 ‘for the discovery of penicillin and its curative effect in various infectious diseases’; the award of the Prize so soon after the discovery of penicillin and its antibiotic effects testifies to the monumental importance of this work.
The large-scale production of penicillin continued to develop during the Second World War with the assistance of the USA, particularly the Rockefeller Foundation. Assisted by the Department of Agriculture’s Northern Regional Research Laboratory in Illinois, Florey was able to substantially increase penicillin production with the use of deep fermentation tanks, the addition of corn steep liquor to the fermentation broth, and the minimisation of acid degradation by controlling pH. Commercial production of penicillin was developed sequentially by Merck, Squibb, Pfizer, Abbott Laboratories, Eli Lilly, Parke Davis, Upjohn, and Wyeth laboratories, eventually leading to the large-scale national production of penicillin.
5.1.1.1 Structure elucidation
In 1943, the British and US governments, appreciating the clinical importance of penicillin, especially in the treatment of battlefield wounds, imposed a ban on the publication of all chemical research on penicillin. Negotiations between the two governments aimed to ensure the complete and confidential exchange of information between the various research groups on both sides of the Atlantic. Much of the work performed in the early stages of penicillin discovery and development was not published at the time, but was told later through personal histories.
The initial attempts at the elucidation of the structure of penicillin were performed by Robert Robinson at the Dyson Perrins laboratory in Oxford, and by 1942 his team had combined their efforts with those of Florey. The low purity of the penicillin initially obtained (approximately 50%) severely hindered the structure determination. An X-ray crystallographic study of penicillin by Squibb Laboratories (USA) identified a structural difference from the penicillin which had been obtained in the UK, and this was later shown to be due to differences in the amide side chains (at position 6) of the two samples of penicillin. The UK sample was 2-pentenylpenicillin (latterly named penicillin F; R1 = MeCH2CH=CHCH2 in the general structure in Figure 5.1.1), while the US sample was penicillin G, or benzylpenicillin. In addition to penicillins F and G, five more side-chain variants were identified in the same year, 1943. Two possible structures were proposed for penicillin: a five-membered oxazolone ring joined to a thiazolidine ring, as reported in the famous Oxford report PEN103 (Abraham et al., 1943) or (correctly) a β-lactam ring fused to the thiazolidine ring (Figure 5.1.5). With the assistance of Dorothy Hodgkin2 and X-ray crystallography, the whole structure was eventually established in 1945 and the presence of the four-membered highly labile β-lactam ring was confirmed.
A vast number of semi-synthetic penicillin antibiotics have been made (outlined in Subsection 5.1.2), which show a range of activities and efficacies; Table 5.1.1 shows the small number still remaining in clinical use. These agents have been limited by resistance to their action as inhibitors of cell-wall synthesis; we will look at this problem in more detail in Subsection 5.1.5.
5.1.1.2 Cephalosporins
Encouraged by the discovery and success of penicillin and other antibiotics discovered from microbial sources, Guiseppe Brotzu, who was Professor of Hygiene at the University of Cagliari in Italy, isolated Cephalosporium acremonium3 from Sardinian sewers in 1945 and showed that this mould inhibited the growth of typhoid bacilli on agar plates. Brotzu had limited resources to continue his studies, but instead sent a culture of the mould, along with a copy of his notes, to Oxford, where Chain and Florey continued the work with the isolation of two antibiotic agents, one of which was identified as a new type of penicillin, while the other was a different kind of agent, a ‘cephalosporin’. A few years later, Edward Abraham and Guy Newton, in the same Oxford laboratory, discovered another cephalosporin from C. acremonium, naming it cephalosporin C. By this time, resistance to penicillin had begun to limit its clinical use in the treatment of staphylococcal infections, but cephalosporin C showed a broad spectrum of antibiotic activity and was effective in protecting mice from penicillin-resistant staphylococcal infections, raising interest in this new class of antibiotics (Abraham and Newton, 1956). The structure of the cephalosporins was again confirmed by Dorothy Hodgkin using X-ray crystallography and was found to include the same β-lactam ring, in this case fused to a dihydrothiazine ring (Hodgkin and Maslen, 1961). A huge number of semi-synthetic cephalosporins have been made, each with their own spectrum of activity; referring back to Table 5.1.2, you can see that their names start with ‘ceph’ or ‘cef’ and they can be classified into ‘generations’:
- Firstgeneration Vary in their spectrum of activity, but are all inactivated by β-lactamase, a class of enzyme found in resistant bacteria and discussed in depth in Subsection 5.1.5.
- Secondgeneration More stable to β-lactamases and have greater activity across Gram negative bacteria. Some can penetrate into the CNS, so are of value in treating meningitis.
- Thirdgeneration Greater β-lactamase stability, a wider spectrum of activity, the ability to cross the meninges, and may be used for surgical prophylaxis.
- Fourthgeneration Resistant to a wide range of β-lactamases, excellent activity against Gram positive and Gram negative bacteria, including Pseudomonas aeruginosa and bacteria resistant to other classes of antibacterial agent.
5.1.1.3 Carbapenems
The carbapenem thienamycin was discovered much later by the Merck laboratories, with its initial isolation from Streptomyces cattleya in 1976 (Kahan et al., 1979). As you will have spotted from Figures 5.1.1–5.1.3, the major difference between the penicillins, cephalosporins, and carbapenems is the position of the sulfur atom and the unsaturation between C2 and C3. Although other naturally occurring carbapenems have been discovered, thienamycin is the most potent, with high antibiotic activity over a broad spectrum of aerobic and anerobic bacteria, both Gram positive and Gram negative, including pencillin-resistant species. It is, however, unstable to a wide range of chemical conditions, contributing to low yields from S. cattleya fermentation, despite considerable work to optimise the conditions. Successful synthetic carbapenems, with wide antibacterial activity, have since been developed and are discussed in Subsection 5.1.2.
5.1.1.4 Monobactams
As we have already seen, the discovery of the penicillins sparked enormous interest in the discovery of other antibiotics. In 1978, to facilitate the testing of large numbers of compounds, the Squibb Institute for Medical Research developed a high-throughput screening programme, which involved a strain of Bacillus lichenformis engineered to be very sensitive to β-lactam antibiotics, to search for new β-lactam agents. Three years later, over 1 million bacterial isolates had been screened and seven monocyclic β-lactams, given the family name monobactams, had been isolated and identified (Figure 5.1.6) (Sykes et al., 1981). These natural monobactams, including SQ 26 445 and SQ 26 180, have only limited antibacterial properties, but structure–activity studies, requiring the synthesis of many chemically modified monbactams, led to aztreonam: the only monobactam used clinically.
Figure 5.1.6 Monobactams: SQ 26 445 (from a strain of Gluconobacter) and SQ 26 180 (from Chromobacterium violaceum) (Sykes et al., 1981)
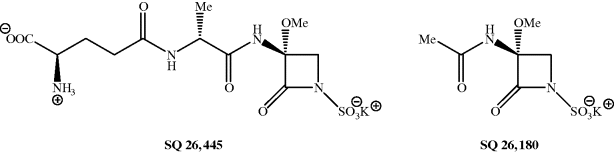
5.1.2 Synthesis
5.1.2.1 Penicillins
The presence of a very labile ring and three stereogenic centres (with only the diastereoisomer shown in Figure 5.1.1 having biological activity) means that the penicillins present a significant synthetic challenge. The first total synthesis to give a penicillin (in this case penicillin V) in any significant quantity was achieved by Sheehan and Henerey-Logan in 1959 (having started this work in 1948!) (Scheme 5.1.1) (Sheehan and Henerey-Logan, 1959). Some notable features of this synthesis are:
- The formation of the thiazolidine ring, from the coupling of D-penicillamine and the malonaldehyde half-ester, gave only two of the four possible diastereoisomers (these are epimers at the 1′-position).
- Deprotection of the amino group, followed by amide bond formation and hydrolysis of the tertiary-butyl ester, gave the precursor to the fused ring system.
- Ring closure to the β-lactam was accomplished with dicyclohexylcarbodiimide (DCC), a reagent commonly used in amide bond formation.
Such total syntheses of penicillins were never going to be able to provide sufficient quantities for clinical use (the overall yield for the steps shown in Scheme 5.1.1 is 1.4%), so a combination of fermentation and organic synthesis was quickly employed to produce a wide range of penicillins. The β-lactam ring is essential for the antibacterial activity of these agents, while structure–activity relationships have shown that any variations in the groups attached to the fused β-lactam-thiazolidine ring structure or the stereochemistry at the 3-, 5-, or 6-positions (highlighted in the box in Figure 5.1.1), leads to a decrease in antibacterial activity. As a result, as you can see from Table 5.1.1, the penicillins usually differ only in the nature of the amide side chain (R1) at position 6. A useful synthetic precursor is, therefore, 6-aminopenicillanic acid, from which a whole range of semi-synthetic penicillins are available by acylation of the amino group (Scheme 5.1.2). An important finding in the early days of penicillin production was that this key intermediate can be obtained by fermentation in the absence of a monosubstituted carboxylic acid (R1COOH) or through the enzyme-catalysed hydrolysis of the 6-amide group of benzylpenicillin (penicillin G) by an acylase (amide hydrolase) (Scheme 5.1.2). The relatively straightforward synthetic procedure of acylation (addition of R1CO) was then used to obtain a range of penicillins.
It’s reasonable to ask here why such a range of penicillins is required, as surely their very similar structures will mean that their properties, including their antibacterial spectrum, as well as any bacterial resistance to them, will be almost identical. This is not actually the case and there are a number of reasons why the availability of a range of penicillins is desirable, including:
- Having a number of possible routes of administration Penicillins such as dicloxacillin, flucloxacillin, phenoxymethylpenicillin, and amoxicillin have increased acid stability, which makes them most suited for oral administration (we will look at the effect of acid, such as that encountered in the stomach, when we look at bioavailability in Subsection 5.1.3). Other penicillins, such as the highly aqueous soluble piperacillin and ticarcillin, have been developed for injection alongside β-lactamase inhibitors to allow rapid treatment of life-threatening infections, such as septicaemia and peritonitis.
- Enhancing bioavailability and decreasing side effects Prodrug esters, such as pivmecillinam, can be employed to enhance the absorption of drugs which have poor oral bioavailability due to their zwitterionic (doubly charged) nature, which results in them being poorly absorbed from the stomach. We will also consider this further in Subsection 5.1.3.
- Having agents with different antibacterial spectra Variation of the C-6 amide R1 group can be used to obtain penicillins with a different antibacterial spectrum; for example, piperacillin has an extended spectrum of activity (including activity against Gram positive and negative organisms, aerobic and anaerobic), while ticarcillin is active only against Gram negative organisms and can be used in the treatment of infections due to P. aeruginosa.
5.1.2.2 Cephalosporins
What really makes the β-lactams exceptional as antibacterial agents (aside from the history of their discovery) is the number of them which are in clinical use. Like the penicillins, there are a number of cephalosporins in clinical use, each of which has its own spectrum of antibacterial activity and physicochemical properties. Once again, a total synthesis would not enable sufficient quantities of the individual antibiotics to be prepared, so these agents are also semi-synthetic and can be obtained from 7-aminocephalosporanic acid (7-ACA). In this case, however, the amino precursor to the cephalosporins cannot be hydrolysed by an acylase, or obtained via fermentation, and so chemical degradation of the amide side chain of the fermentation product, cephalosporin C, is employed (Scheme 5.1.3) (Campos Muñiz et al., 2007).
Scheme 5.1.3 Preparation of 7-aminocephalosporanic acid (7-ACA) from cephalosporin C, involving protection of the carboxylic acids and amine, conversion of the secondary amide to an imidoyl chloride and then an imidate, and hydrolysis of the imidate
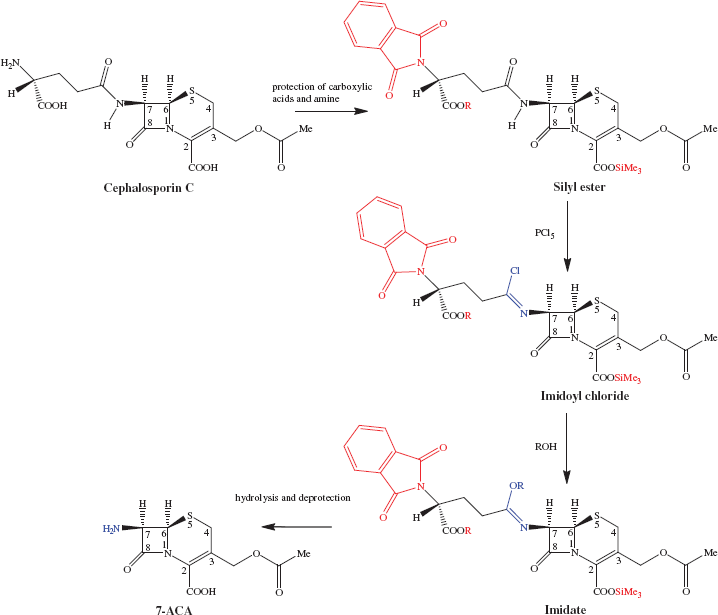
This is not quite as easy as it might seem, as the chemical hydrolysis of the side-chain secondary amide must be accomplished in the presence of a highly strained cyclic tertiary amide and a chemically labile ester group. Harsh methods, such as those involving acid or base reflux, cannot be used and the hydrolysis therefore has to take advantage of a unique property of the secondary amide. The chlorination of amides with inorganic chlorides, such as PCl3, PCl5, or SOCl2, gives rise to a very reactive class of compounds known as imidoyl chlorides, in which a reactive Cl-C=N group replaces the amide bond. Formation of an imidoyl chloride is possible for the side-chain secondary amide, but not for the β-lactam, and this is the basis for the chemoselectivity4 of this process. The imidoyl chloride is then reacted with an alcohol to form an imidate, which is readily hydrolysed to an amine (in this case, 7-ACA) and an ester (Scheme 5.1.3). In a similar manner to 6-APA, 7-ACA can then be acylated to give the first- to fourth-generation cephalosporins. The possibility of using enzymes to carry out this conversion has also been investigated (Parmar et al., 1998).
5.1.2.3 Carbapenems
As you can see from Table 5.1.3, there are only four carbapenems, but, like the penicillins and cephalosporins, each has an individual spectrum of activity and properties, making it distinct from the rest of the group. The carbapenems have remained active against a wide range of β-lactam-resistant bacteria and, except for ertapenem, are active against the notoriously treatment-resistant P. aeruginosa, resulting in an important position for these members of the antibiotic armoury (although recent developments are threatening this position: read on to Subsection 5.1.5). We met the natural carbapenem thienamycin in Subsection 5.1.1.3; although highly potent and broad-spectrum, its instability prevents its clinical use. One major limiting problem is due to ring-opening of the β-lactam ring of thienamycin by the nucleophilic side-chain amino group of another thienamycin molecule. Except for thienamycin itself, there is no naturally available intermediate for the semi-synthesis of carbapenems, and as a consequence, despite the structural complexity and the functional group sensitivity, the production of thienamycin analogues has been pursued via synthetic chemistry, perhaps explaining why there are so few of these β-lactam antibiotics available. Imipenem, the first synthetic clinical carbapenem, was prepared from thienamycin (refer to Table 5.1.3 and Figure 5.1.3 for the structures); it seems remarkable that the minor structural change between thienamycin and imipenem should make such a difference, yet imipenem is significantly more stable with many clinical applications. The basicity of the amidine group, which is protonated at physiological pH, results in the loss of the side chain’s nucleophilic properties and greater stability of the β-lactam ring (Kesado et al., 1980). Unfortunately, imipenem was found to cause nephrotoxicity and must be co-administered with an inhibitor (you can read more about this in Subsection 5.1.6); in the later carbapenems – meropenem, ertapenem, and doripenem – a methyl group at C1 essentially eliminates this problem. Most syntheses of carbapenems rely on the intramolecular condensation of monocyclic β-lactam 1, in which the α-keto ester group reacts with the β-lactam nitrogen atom to give the key intermediate 2 (Scheme 5.1.4).
Scheme 5.1.4 Key intramolecular condensation step in the synthesis of carbapenems (Prashad et al., 1998)
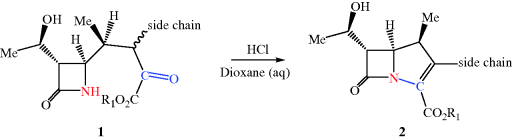
5.1.2.4 Monobactams
No intermediates are available for the semi-synthesis of aztreonam, and total synthesis is the only option. Despite appearing to be relatively simple, aztreonam has an unusual functional group (an acyl sulfamate) and two stereogenic centres, and so even a relatively simple synthetic route, such as that by the Squibb group, has five steps (Scheme 5.1.5) (Cimarusti et al., 1983). You’ll notice that this route again requires a protecting group and a mesylate group (MeSO3), which acts as the leaving group in the cyclisation to the azetidinone. The final step involves fairly routine amide-bond-forming conditions, with DCC (see Scheme 5.1.1) and 1-hydroxybenzotriazole (HOBt).
5.1.3 Bioavailability
The β-lactam antibiotics are time-dependent agents, which means that their clinical success is influenced predominantly by the length of time for which the drug concentration is maintained above the minimum inhibitory concentration (MIC) at the site of infection (T > MIC) (MacGowan, 2011). The penicillins and cephalosporins have a limited post-antibiotic effect (PAE) on Gram negative bacteria, but a longer effect of 1.2–7.1 hours on Gram positive bacteria and H. influenzae (MacGregor and Graziani, 1997). The carbapenems, by contrast, exert a PAE of several hours against both Gram positive and Gram negative bacteria (Mouton et al., 2000). Whatever the β-lactam agent and infecting organism, though, the T > MIC parameter is the major consideration and it is believed that maintaining the antibiotic concentration above the MIC for >60% of the dosing interval is optimal for therapeutic success in the clinic (Turnidge and Paterson, 2007).
The carboxylic acid group that is integral to the β-lactam antibiotics allows their formulation as a salt, most often as the sodium salt, suitable for infusion or injection, which is a positive feature of these agents. In fact, some β-lactam antibiotics (e.g. piperacillin, ticarcillin) have been developed specifically for IV and intramuscular (IM) administration. Oral administration, however, offers a wider flexibility of application, is usually more economically favourable, and is generally more acceptable to patients. Oral administration of many of this class of agents (especially the penicillins, e.g. benzylpenicillin and meticillin) is limited by their acid-sensitivity – they are inactivated in the stomach by acidic hydrolysis, which opens up the β-lactam ring and renders them inactive. The extent of inactivation is sufficient to reduce the bioavailability significantly, and other penicillins were developed in order to overcome this problem and facilitate oral use. Consider the functional groups present in even a simple example β-lactam: benzylpenicillin contains carboxylic acid, thioether, and amide groups, none of which are renowned for being particularly sensitive to acid, so what is it about this molecule that makes it prone to acid hydrolysis? Well, the relatively unusual four-membered lactam ring should grab your attention, as it this which introduces a high degree of strain to the molecule:
- First, you will remember that the normal bond angle for an sp2 hydridised carbon (as found in a carbonyl group) is around 120°, yet in the β-lactam ring it is close to 90°; even the sp3 hydridised carbon atoms in this ring have a reduced bond angle (from 109° to 90°). We can conclude that this ring amide carbonyl suffers from angle strain.
- Second, as a four-membered ring has little flexibility, the substituents on each ring atom are partly eclipsed,5 which adds torsional strain to this system.
- Third, in linear molecules, amide carbonyls are stabilised by resonance with the N lone pair (which is part of the reason for the usual stability of amide bonds6), but this cannot happen effectively in this cyclic amide as the fused thiazolidine ring restricts the possible conformations of this molecule, such that it adopts a wide V-shape and the N is not able to delocalise its electrons into the carbonyl π-orbital.7 Flattening the bicyclic structure to allow resonance to occur would form an impossibly strained system (Figure 5.1.7). Additional steric strain caused by the bicyclic system leads to increased chemical instability of the carbonyl group.
- One more structural aspect adds to the lack of stability to acid: the side-chain amide group attached to the β−lactam ring. This group can attack the ring amide carbonyl C, causing opening of the four-membered ring and the eventual formation of two products: penillic acids and penicillenic acids (Scheme 5.1.6). The reaction is promoted under acidic conditions and is the major reason for the decomposition of some penicillins in the stomach after oral administration.
The first three of these structural features are inherent to all penicillins, so cannot be changed without altering the potency (usually with a drastic reduction in or total loss of activity), and are of lesser importance to acid stability anyway. The contribution from the final, more major, problem can be reduced by forming penicillin analogues with an electron-withdrawing group on the amide side chain at C-6; the attack by this group on the ring carbonyl is then suppressed and these β-lactams are sufficiently stable to acidic conditions for oral administration. Phenoxymethylpenicillin (penicillin V) (Figure 5.1.8) has a more acid-stable structure than benzylpenicillin – you can see that they only differ by an O atom as part of the R1 side chain, which exerts enough electron-withdrawing effect on the side-chain amide carbonyl of penicillin V to inhibit the cyclisation on to the ring carbonyl group (Scheme 5.1.6). This increases the half-life for stability in acid from about 30 minutes for benzylpenicillin to more than 5 hours for phenoxymethylpenicillin (Barza and Weinstein, 1976), thus allowing more than enough time for it to be absorbed from the gastrointestinal (GI) tract. Although penicillin V has a lower potency than pencillin G, its greater bioavailability after oral administration allowed it to largely replace the penicillin G injections in clinical use in the late 1950s (Hart et al., 1956). Similarly, steric bulk in the side chain suppresses cyclisation; an example is amoxicillin, and this penicillin is also suitable for oral administration.
Figure 5.1.8 Electron-withdrawing substituents on the 6-amino group of penicillin inhibit attack of the linear amide carbonyl group on the ring amide carbonyl carbon and enhance acid stability
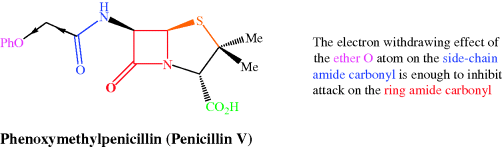
Another barrier to oral bioavailability is that of low lipophilicity seen in particularly polar molecules with a low logP value – these molecules are usually poorly absorbed across biological membranes and suffer from low bioavailability. As β-lactam antibiotics remaining in the GI tract can kill commensal bacteria8 and cause diarrhoea, it is obviously something to avoid. Most β-lactam antibiotics have only one ionisable group, the carboxylic acid. However, some have more than one group that can ionise at the various pH values seen in the GI tract, and these suffer from particularly poor absorption across the gastric mucosa.
Consider the structure of ampicillin in Figure 5.1.9: how many ionisable groups are there in this molecule, where are they, and at what pH values are they ionised? Is there a pH value at which ampicillin is un-ionised?
We have already considered the functional groups that are found on all penicillins: the ring amide, side-chain amide, thioether, and carboxylic acid groups. The only extra group in ampicillin is an amine on the R1 side chain. Of these groups, you will have spotted that only the carboxylic acid (pKa 2.5) and amine (pKa 7.2) are ionisable at the pH range found in the GI tract. If we construct acid–base equilibria for ampicillin (as we did for the aminoglycosides) at the approximate pH values it would encounter after oral administration, we can work out its charge in each environment (Scheme 5.1.7).
As the equilibria show, ampicillin is ionised and highly polar at every location in the GI tract, particularly in the duodenum, where it exists as a zwitterion; not surprisingly, it has low oral bioavailability. Yet ampicillin, and other β-lactam antibiotics that have similarly ionisable groups, have very useful antibacterial activity and so are important members of this class. One solution to this problem has been the use of prodrugs.9 Of the many possible prodrug groups for carboxylic acids (Rautio et al., 2008), esters are most commonly used to overcome the physicochemical problems presented by carboxylate-containing pharmaceutical products (Beaumont et al., 2003), as the almost ubiquitous distribution of esterases in the body provides a reliable and rapid method for the release of the active parent drug; ester prodrugs are hydrolysed mostly by serum esterases after absorption from the GI tract. Usually, a simple ester, like an ethyl group, is satisfactory, but in this case the ethyl ester of ampicillin, and of other β-lactams, is not a good esterase substrate; although it is often claimed that the size and shape of the penicillin provides steric hindrance and prevents the ester group from accessing the active site of the esterase for efficient hydrolysis, it has been shown that the simple esterified penicillins are rapidly degraded by attack at the β-lactam ring (Nielsen and Bundegaard, 1988). A double-ester approach was developed to overcome this problem and has been successfully applied to a wide range of drugs, including the β-lactam antibiotics. Examples can be found in Table 5.1.1: pivmecillinam and cefpodoxime proxetil, along with others found in clinical use, such as pivampicillin and cefuroxime axetil. Taking pivmecillinam as an example, we can see that the carboxylate of mecillinam has been derivatised by an extended double ester, called an α-acyloxyalkylester: the first ester group is formed by the derivatisation of mecillinam’s carboxylate group, while the second ester group is removed from the penicillin by several atoms’ distance, which facilitates access to the esterase and enables hydrolysis of the second ester group to form an unstable intermediate (Scheme 5.1.8).
Scheme 5.1.8 Esterase hydrolysis of pivmecillinam, an α-acyloxyalkyl ester (double ester), through an unstable intermediate that spontaneously decomposes to release the active antibiotic
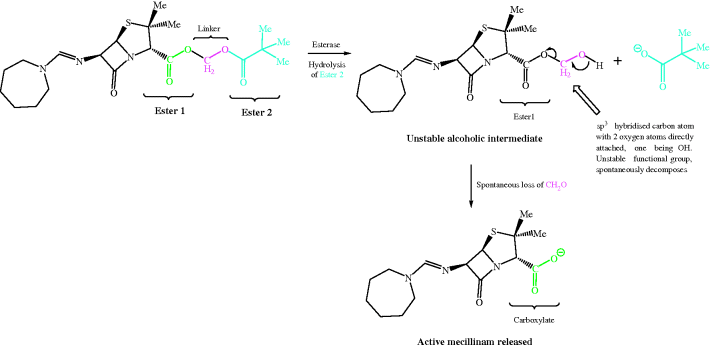
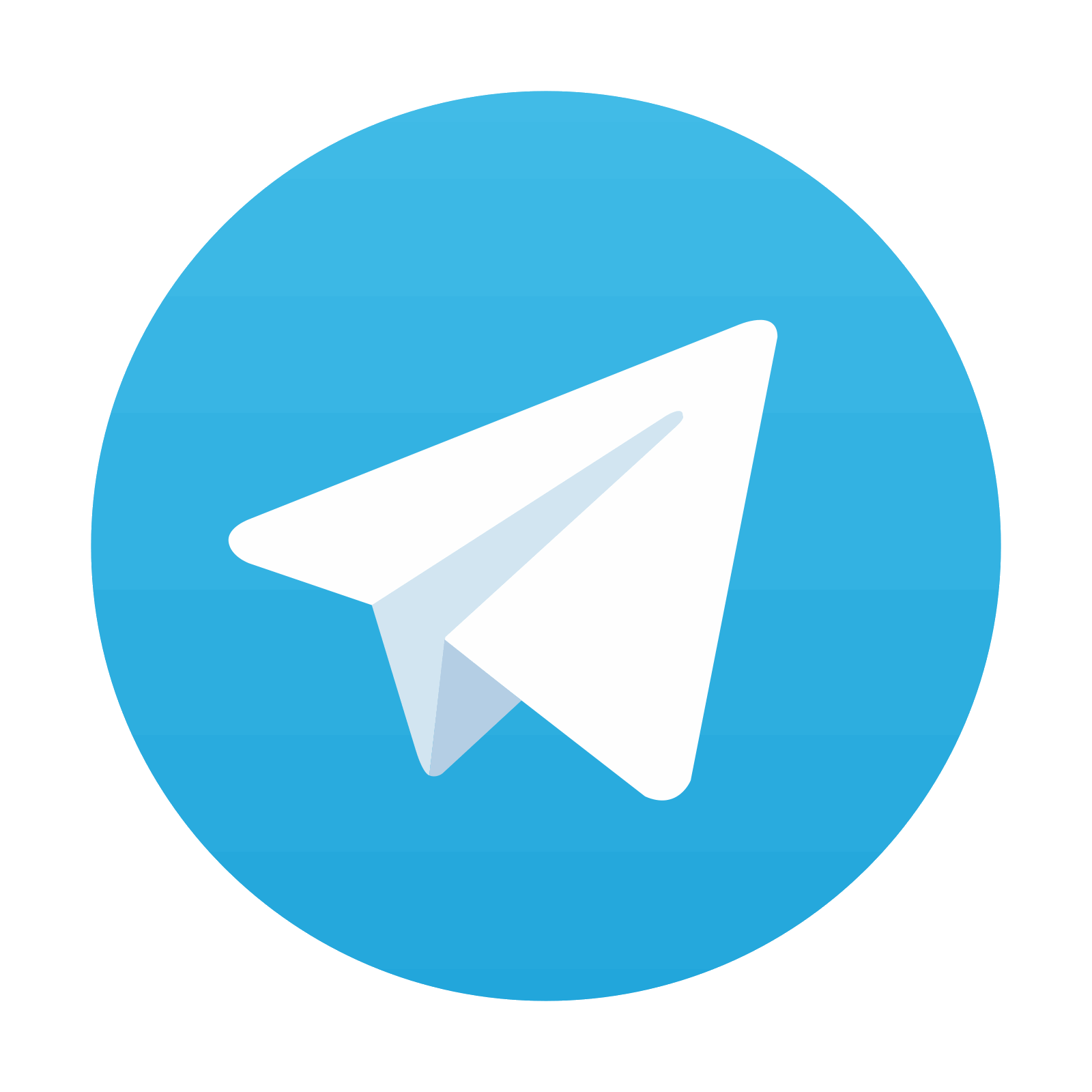
Stay updated, free articles. Join our Telegram channel
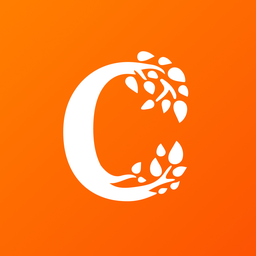
Full access? Get Clinical Tree
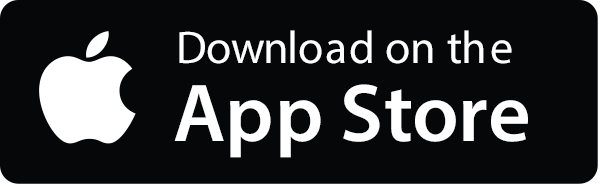
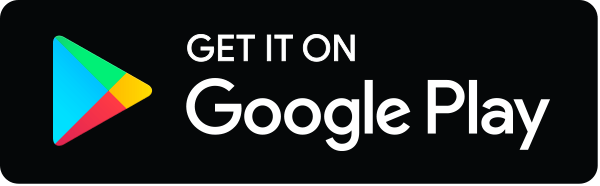