Type 1 and 5 secretion systems and associated toxins
Timothy J. Wells and Ian R. Henderson, University of Birmingham, Birmingham, UK
Introduction
Pathogenic Escherichia coli can cause disease in a variety of locations in the human body. The site of infection is dependent on the repertoire of virulence factors such as adhesins and toxins expressed by the pathogen. Most proteins involved in virulence need to traverse both the inner and outer membrane of the bacterium and in some cases the membrane of the host cell to perform their function. Given the diversity of function, multitude of targets and variety of structures of these secreted proteins, it is unsurprising to find that E. coli has multiple different secretion systems to effect translocation of proteins to the exterior of the cell.
Two of the simplest secretion systems that E. coli utilizes are the type 1 secretion system (T1SS) and the type 5 secretion system (T5SS). These secretion systems are widely distributed in other Gram-negative bacteria. In E. coli the best-known T1SS is that used to secrete hemolysin, a pore-forming toxin found in both uropathogenic (UPEC) and enterohemorrhagic (EHEC) strains of E. coli. The T5SS is widespread in E. coli with many of the virulence factors secreted by this mechanism specific for a particular pathotype. This chapter will focus on the T1SS and T5SS; the mechanism of biogenesis, the function of the secreted proteins and current attempts to use these systems in biotechnological applications. Other E. coli secretion systems are discussed in detail in other chapters.
The type 1 secretion system
The T1SS of Gram-negative bacteria allows the secretion of proteins from the cytoplasm to the extracellular environment in a single step, with no periplasmic intermediate (Figure 16.1). Proteins secreted by the T1SS greatly vary in size, from 82 amino acids (aa) to over 8000 residues (Delepelaire, 2004). The secreted proteins are diverse in function and include toxins, proteases, lipases, S-layer proteins, hemophores, bacteriocins, and others with as yet unknown functions (Delepelaire, 2004). Although divergent in sequence, the majority of proteins secreted by the T1SS have a C-terminal located secretion signal, which is recognized by the translocation machinery; the exception is dispersin of EAEC which is secreted to the periplasm by the Sec system before secretion via the T1SS to the extracellular milieu (Koronakis et al., 1989; Ghigo and Wandersman, 1994; Sheikh et al., 2002).
The translocating machinery of a T1SS is comprised of three proteins that span the cell envelope, all of which are essential for secretion (Letoffe et al., 1996). Two of the translocation proteins span the inner membrane (the ATP-binding cassette [ABC] and the membrane fusion/adapter protein [MFP]), while the final member of the translocator is an outer-membrane protein (OMP) (Figure 16.1). The ABC protein possesses a nucleotide-binding domain (NBD) fused to a transmembrane domain (TMD), and recognizes the C-terminal secretion signal of the substrate molecule; as such this protein is responsible for the specificity of the secretion machinery for the substrate molecule (Delepelaire, 2004). The MFP protein consists of a short cytoplasmic domain at the N-terminus, followed by a membrane anchor and a large periplasmic domain (Dinh et al., 1994; Thanabalu et al., 1998). The OMP forms a trimeric, water-filled channel through the outer membrane, open to the extracellular milieu but constricted at the periplasmic end (Koronakis et al., 2000). The interaction of the secreted effector molecule with the ABC protein triggers the sequential assembly of the secretion complex by generating interactions between the ABC, MFP, and OMP (Thanabalu et al., 1998). When the MFP protein binds the OMP, it triggers the opening of the periplasmic end thus creating a complete channel from the cytoplasm to the extracellular medium (Thanabalu et al., 1998). Hydrolysis of ATP by the ABC protein provides the energy for the secretion of the substrate protein through the channel (Figure 16.1).
Proteins secreted via the T1SS can have diverse functions, however virtually all T1SS proteins found in E. coli belong to the repeat-in-toxin (RTX) family; the exception is dispersin of EAEC (Linhartova et al., 2010). RTX proteins are so named for the distinctive glycine-rich repeats (GGXGXDXXX) that specifically bind calcium (Welch, 2001). All RTX toxins are made as an inactive precursor and must undergo a common post-translational maturation step before export by the T1SS (Linhartova, et al., 2010). RTX family members can have a variety of functions, however the prominent and historically the first described group consists of toxins exhibiting a cytotoxic pore-forming ability, often first detected as a hemolytic halo surrounding bacterial colonies grown on blood agar plates (Goebel and Hedgpeth, 1982; Muller et al., 1983; Felmlee et al., 1985; Welch, 1991). RTX proteins found in E. coli include TosA from UPEC strains needed for adherence and colonization of the urinary tract (Parham et al., 2005; Iguchi et al., 2009; Lloyd et al., 2009; Vigil et al., 2011a,b, 2012), EhxA from EHEC strains (Bauer and Welch, 1996) and the prototypical member of the T1SS, hemolysin (HlyA), from uropathogenic E. coli.
Hemolysin
Regulation and maturation
Hemolysin of UPEC was the first described T1SS protein. Hemolysin is an important virulence factor in E. coli infections (see Chapter 9) owing to its cytolytic and cytotoxic activity against a wide range of mammalian cell types (Welch et al., 1981). The synthesis, activation and secretion of hemolysin is determined by the hlyCABD operon (Hess et al., 1986). In E. coli this operon is located either on chromosome-bound pathogenicity islands or on transmissible plasmids, suggesting that the T1SS has been acquired by horizontal gene transfer. The operon encodes the toxin activation protein (HlyC), the hemolysin itself (HlyA), the ABC transporter (HlyB), and the MFP protein (HlyD). The outer-membrane component, the multifunctional protein TolC, is found in all E. coli strains and is encoded elsewhere on the chromosome.
Full transcription of the hlyCABDoperon is reliant on two factors, the 18-kDa protein RfaH (Bailey et al., 1992) and a motif 2-kb upstream from the operon termed the ops (operon polarity suppressor) (Bailey et al., 1996; Nieto et al., 1996; Leeds and Welch, 1997). RfaH is a bacterial elongation factor that increases expression of distal genes in several long, horizontally acquired operons, including those encoding lipopolysaccharide (LPS) and F (fertility) pilus biogenesis (Bailey et al., 1997). Loss of RfaH or the ops element shows a distinctive effect on hlyCABD transcription, modestly decreasing transcription of the first two genes, hlyC and A, but virtually abolishing transcription of hlyB and D (Bailey et al., 1992, 1996; Nieto et al., 1996). The ops element is required for RfaH recruitment to RNA polymerase (RNAP) (Bailey et al., 1996; Artsimovitch and Landick, 2002; Belogurov et al., 2009); it induces isomerization of the transcription elongation complex (TEC) into a distinct state necessary for the sequence-specific recruitment of RfaH to the non-template DNA strand. Following recruitment, RfaH remains bound to RNAP and acts as an antiterminator by reducing RNAP pausing and termination at some factor-independent and Rho-dependent signals (Bailey et al., 1996).
After translation of HlyA, the protein is inactive until matured intracellularly by the cotranslated HlyC, a fatty acid acyltransferase (Issartel et al., 1991). HlyC forms a homodimer that uses acyl–acyl carrier protein (ACP) as the fatty acid donor to acylate two internal lysine residues, K564 (K1) and K690 (KII) of HlyA. Although both lysines are acylated independently, both are required for in vivo toxin activity. However, acylation is only required for the hemolytic activity; the secretion of HlyA is independent of HlyC (Koronakis and Hughes, 1996).
After acylation HlyA is secreted via a T1SS formed by the proteins HlyB, HlyD, and TolC. HlyB, the ABC component of secretion, couples ATP hydrolysis to the secretion of HlyA (Koronakis et al., 1995). The topology of HlyB (707 aa) has been determined, suggesting that the protein is inserted in the inner membrane by eight hydrophobic, α-helical transmembrane domains (TMDs) extending from the amino acid positions 38–432 (Wang et al., 1991). The cytoplasmic loops of HlyB are large with many positively charged amino acids, whereas in contrast the periplasmic loops are quite small. HlyD is a prototypical and well-characterized member of the MFP family (Dinh et al., 1994). HlyD is anchored in the cytoplasmic membrane by a single TMD and possesses a large 100 aa C-terminal periplasmic domain (Schulein et al., 1992). This periplasmic domain is highly conserved not only within the MFP family but also within the superfamily of periplasmic efflux proteins (PEP) (Schulein et al., 1992). Contact between the HlyB:HlyD inner membrane complex and the final component, TolC in the outer membrane, is primarily mediated by HlyD in response to engagement by the HlyA substrate (Thanabalu et al., 1998). The fully active complex contains the substrate and all three export proteins, all of which undergo conformational change. The complex is transient however; once the substrate leaves the cell the inner-membrane and outer-membrane components disengage (Thanabalu et al., 1998). As TolC is involved with at least four different export systems, the mechanism behind forming the physical bridge across the periplasm has been well studied.
Secretion of HlyA via the TolC channel
TolC (55 kDa) is a multifunctional OMP in E. coli that can serve as an outer membrane component for several processes, including T1SS and drug export via RND (resistance, nodulation, division) systems (Wandersman and Delepelaire, 1990; Zgurskaya and Nikaido, 2000). Crystal structures of TolC reveal that it is a homotrimer in the outer membrane of E. coli where it forms a trans-periplasmic channel-tunnel. The trimer is about 140 Å in length, comprising a 40 Å β-barrel (channel domain) and a 100 Å long α-helical barrel that projects across the periplasmic space (tunnel domain) (Koronakis et al., 2000). The molecule forms a tapering tube that is almost closed at the periplasm end and wide open at the outer-membrane surface. The internal diameter of the channel is around 35 Å and consists of a large water-filled cavity open to the extracellular medium (Koronakis et al., 2000).
It is proposed that TolC opens at the proximal end by an iris-like mechanism, by unwinding the coiled-coiled helices so as to move the inner ring to the exterior. This would open the periplasmic end of the channel going from 5 Å in diameter to a maximal opening of around 20 Å (Figure 16.2) (Koronakis et al., 2000; Bavro et al., 2008). Crystal structures of TolC mutants either stuck in closed or semi-open states support this hypothesis (Bavro et al., 2008). Opening of TolC is triggered by members of the MFP class (HlyD in the case of hemolysin) (Janganan et al., 2011). Thus, HlyA binds HlyB, triggering a change in conformation in the HlyB/HlyD complex. HlyD then binds to TolC, triggering the opening of the periplasmic end of the TolC channel and making a complete pore from cytoplasm to extracellular medium. The HlyA protein is then secreted through the pore to the extracellular medium.
FIGURE 16.2 The structure of trimeric TolC modeled in the closed and open positions. The trimeric protein is made of a 40 Å channel domain in the outer membrane and a 100 Å tunnel domain in the periplasm. Binding of the MFP protein initiates opening of the TolC periplasmic domain by an iris-like movement enlarging the tunnel diameter from 5 Å to 20 Å. Figure adapted from Protein Data Bank (Bavro et al., 2008).
Mechanisms of action and role in virulence
Once secreted, E. coli HlyA acts as a pore-forming RTX cytotoxin, with a broad range of cytocidal activity on a wide spectrum of cells from a variety of species, including erythrocytes, leukocytes, granulocytes, monocytes, endothelial cells, and renal epithelial cells (Gadeberg and Orskov, 1984; Keane et al., 1987; Bhakdi et al., 1989, 1990; Mobley et al., 1990; Suttorp et al., 1990). HlyA alteration of membrane permeability causes lysis and death, which may provide iron and prevent phagocytosis. Once inserted, HlyA behaves as an integral membrane protein and causes target cell lysis by forming transmembrane pores that are cation-selective, pH-dependent, and apparently asymmetric (Bhakdi et al., 1986; Menestrina et al., 1995). Membrane insertion of HlyA is thought to be through a monomolecular mechanism. It has been estimated that only 1–3 HlyA molecules form the pore, with oligimerization occurring by subsequent addition of monomers within the membrane (Bhakdi et al., 1986; Benz et al., 1989). A conserved region toward the N-terminus of HlyA is essential for lysis and is predicted to be involved in pore formation, as it spans the only pronounced hydrophobic sequences in the otherwise hydrophilic HlyA protein (Stanley et al., 1998). The C-terminal glycine-rich repeat domain that binds Ca2+ is required for hemolysis but not for pore formation, and it seems likely that Ca2+-induced conformational change may promote a subsequent insertion of the toxin into cell membranes (Bakas et al., 1998; Stanley et al., 1998).
In addition to pore formation, at very low, sublytic concentrations, HlyA is a potent trigger of G-protein-dependent generation of inositol triphosphate and diacylglycerol in granulocytes and endothelial cells, stimulating the respiratory burst and the secretion of vesicular constituents (Bhakdi and Martin, 1991). It has also been shown to stimulate the release of cytokines, including interleukin 1β and tumor necrosis factor (TNF) from a variety of human cells (Suttorp et al., 1993). All of these phenotypes suggest that HlyA would be a potent virulence factor in UPEC infections, however defining the actual role that HlyA may play in pathogenicity has been elusive (Linggood and Ingram, 1982; Mobley et al., 1990; Yamamoto et al., 1995; Smith et al., 2006). Recent studies however have shown that HlyA, although not contributing to colonization, does play a major role in evoking damage in the uroepithelium and in inducing hemorrhage in the bladder during the early stages of E. coli-mediated cystitis in the mouse (Smith et al., 2008).
The Type 5 secretion system
The T5SS is the simplest and most widespread protein secretion mechanism in Gram-negative bacteria. The secretion system is currently divided into five subclasses (types 5a to 5e) based on differences in gene organization and protein structure. Type 5a are better known as the classical autotransporters (ATs), type 5b as the two-partner secretion pathway (TPS), and type 5c as the trimeric autotransporter adhesins (TAA). Type 5d have only recently been described (Figure 16.3). The 5e subclass has only been added to the T5SS family recently, but includes the functionally well-characterized Intimin of E. coli and Invasin of Yersinia.
Molecular organization
Autotransporters (ATs) are so named based on the hypothesis that all the functional elements required for secretion are contained in a single protein. Structurally, AT proteins are characterized by the presence of three distinct domains: (i) an N-terminal signal sequence; (ii) a secreted passenger/α-domain; and (iii) a C-terminal domain termed the translocation or β-domain (Jose et al., 1995). The signal-sequence mediates translocation across the inner membrane in a Sec-dependent manner. Once in the periplasm the translocation domain forms a β-barrel composed of 12 β-strands that inserts into the outer membrane (Henderson et al., 1998; Henderson and Nataro, 2001). Finally the β-barrel mediates translocation of the passenger domain to the surface where the secreted polypeptide is either cleaved or remains attached to the bacterium (Henderson et al., 2004). While the translocation domain is highly homologous among AT proteins (conserving the mechanism of transport), the secreted passenger domain demonstrates considerable amino acid sequence variation. ATs can also vary greatly in length, from as low as 500 aa to above 3000 aa.
Two-partner secretion (TPS) is similar to the AT mechanism, requiring a passenger domain and a β-barrel translocation domain. However, instead of being encoded on a single polypeptide, the passenger and translocation domains are produced as two separate proteins, each containing a Sec-dependent N-terminal signal sequence to mediate inner-membrane translocation to the periplasm (Jacob-Dubuisson et al., 2001). These proteins are referred to as TpsA (passenger) and TpsB (translocation) family members (Jacob-Dubuisson et al., 2001) and are frequently, but not always, encoded in an operon. Akin to the AT mechanism, once in the periplasm the translocation β-barrel mediates secretion of the passenger domain (Henderson et al., 2000). Whilst TpsA proteins can have widely differing functions, they share common features; they are large (many >3000 aa), they share significant sequence similarity within their N-terminal 300 aa (a region called the TPS domain) (Jacob-Dubuisson et al., 2001, 2004) and like the AT passenger domain adopt a β-helical conformation. The β-barrel of TpsB proteins is different to AT proteins being composed of 16 amphipathic β-strands instead of the usual 12 found in ATs (Guedin et al., 2000) and containing two polypeptide-transport-associated (POTRA) domains that descend into the periplasm and are thought to recognize the TpsA protein via the TPS domain. The POTRA domains are predicted to recruit nascent β-strands in a process called β-augmentation; in TPS proteins it is thought that the POTRA motif recognizes the TPS domain of TpsA proteins (Knowles et al., 2008; Jacob-Dubuisson et al., 2009; Delattre et al., 2011).
The third subgroup, the trimeric autotransporter adhesins (TAA) are produced as a single polypeptide similar to ATs, however they differ significantly in the size of the translocation domain. Instead of being comprised of ~300 aa forming 12 β-strands, a translocation domain of 70–100 aa is sufficient to mediate secretion of the passenger domain (Roggenkamp et al., 2003; Surana et al., 2004). This shorter translocation domain has been shown to form four β-strands which only become a functional 12-stranded β-barrel by forming a homotrimer in the outer membrane (Cotter et al., 2005; Linke et al., 2006). Trimerization of the passenger domain is vital for stability and functionality of the protein (Cotter et al., 2006). As the name suggests all TAA proteins identified to date have a role in adhesion.
Recently, a patatin-like protein named PlpD was identified in an environmental Pseudomonas strain and described to belong to a fourth sub-group of T5SS (Salacha et al., 2010). Like the classical ATs, the protein is produced as a single polypeptide with an N-terminal signal sequence, a passenger domain and a β-barrel translocation unit, however the β-barrel domain in type 5d is composed of 16 β-strands and more closely related to the TpsB proteins of two-partner secretion. There is also a POTRA motif between the passenger and translocation domains, suggesting a gene fusion event combining the two components of a TPS system. No members of the type 5d have been identified in E. coli and these proteins will not be considered further here.
Finally, Intimin of E. coli (see Chapters 4 and 5) and Invasin of Yersinia, have long been studied for their role in adhesion but due to their vastly different genetic organization they have only recently been identified as belonging to T5SS. The proteins possess an N-terminal signal sequence, but in contrast to classical ATs, the order of the passenger and translocation domains are reversed (Tsai et al., 2010). In addition to this reversal, between the translocation β-barrel and signal peptide is an α-helical region and a LysM domain thought to bind to peptidoglycan (Fairman et al., 2012). The β-barrel, similar to classical ATs, is composed of 12 β-strands (Fairman et al., 2012). The inverted domain structure results in the C-terminus, not the N-terminus being secreted to the cell surface where it extends from the outer membrane to contact the host cell.
Secretion
It was initially hypothesized that T5SS proteins were unique in that all elements required for secretion were found in either one or two proteins. However, recent studies reveal that the reality is more complicated, with periplasmic chaperones and machinery in the inner and outer membranes also required for secretion. The majority of work on T5SS secretion has been focused on classical ATs, so that little is yet known about the requirements of types 5b–e secreted proteins. However as the subgroups have many similarities in structure and domain organization it would be unsurprising if they shared many of the requirements of classical ATs.
All five subgroups of T5SS once translated are targeted to the Sec machinery, which catalyzes their energy-driven transport to the periplasm. Most T5SS proteins are synthesized with characteristic Sec-dependent signal peptides, typically 20–30 aa in length. These signal peptides typically consist of a positively charged N-domain, followed by a hydrophobic H domain and finally a C domain that contains a recognition site for a signal peptidase. On entering the periplasm the signal peptidase recognizes the uncharged residues at –1 and –3 relative to the cleavage site (Hegde and Bernstein, 2006). A significant subset of T5SS proteins (including members of types 5a, 5b, 5c, and 5e) possess unusually long signal peptides, typically 50–60 residues long. The purpose of the longer signal peptide has been studied for multiple proteins with differing results; however the consensus view is that the extended peptide has a subtle role, slowing translocation of the proteins across the inner membrane to prevent accumulation of misfolded species in the periplasm. The precise mechanism governing the decrease in translocation has yet to be elucidated (Leyton et al., 2010, 2012).
On entering the periplasm, all further steps in the secretion pathway must be independent of any energy input. Many OMPs have been shown to interact with periplasmic chaperones such as SurA, Skp, and the bifuncational periplasmic serine protease DegP. Many AT proteins also have been shown to interact with these proteins (Sklar et al., 2007; Baud et al., 2009; Knowles et al., 2009) suggesting that T5SS biogenesis follows the same rules as normal OMPs, whereby these chaperones mediate transit through the periplasm. Chaperones have also been shown to be involved for the other T5SS subtypes, with DegP interacting with the TPS protein FHA (Baud et al., 2009), and Intimin shown to interact with SurA, Skp, and DebP (Bodelon et al., 2009). The role of chaperones is currently unknown for TPS and TAA proteins. Thus, it seems that periplasmic chaperones act specifically to keep the passenger domain in a translocation-competent form and prevent aggregation and mis-folding (Lazar and Kolter, 1996; Leyton et al., 2012).
In addition to chaperones, the β-barrel assembly machinery (Bam) complex has been shown to be required for the biogenesis of many ATs (Ieva and Bernstein, 2009; Ruiz-Perez et al., 2009, 2010). The Bam complex is comprised of the integral β-barrel protein, BamA, and four peripheral lipoproteins, BamB–E (Knowles et al., 2009). BamA and BamD have been shown to be essential for the correct secretion of AT passenger domains across the outer membrane, whereas BamB, C, and E are not required (Rossiter et al., 2011b). However, redundant roles for BamB, C, and E have not been ruled out. BamA consists of a large C-terminal β-barrel in the outer membrane and five N-terminal POTRA domains that descend into the periplasm. POTRA 5 makes direct contact with the lipoprotein BamD (Kim et al., 2007), while POTRA 1 and 2 have been shown to bind peptides of OMPs (Knowles et al., 2008) and the chaperone SurA (Bennion et al., 2010). As SurA interacts with BamA, it is conceivable that ATs are delivered to the Bam complex by periplasmic chaperones. BamA has also been shown to be important for the secretion of proteins belonging to other subgroups of T5SS, including the TPS CdiA/B (Aoki et al., 2008), the TAA YadA (Lehr et al., 2010), and the type 5e secreted Intimin (Bodelon et al., 2009). The role of BamD in TPS, TAA, and type 5e is yet to be elucidated.
Finally, the translocation and assembly module (TAM) was recently described to be required for efficient secretion of ATs in proteobacteria. The TAM consists of two proteins, TamA in the outer membrane and TamB in the inner membrane that together span the entire cell envelope. TamA, like BamA and TpsB proteins discussed above, belongs to the Omp85 superfamily of outer-membrane proteins. It consists of a C-terminal β-barrel in the outer membrane and three N-terminal POTRA domains thought to interact with TamB (Selkrig et al., 2012). Mutants lacking the TAM complex in E.coli failed to secrete the type 5a adhesins Antigen 43 and EhaA (Selkrig et al., 2012). It is as yet unknown if the TAM complex is required for efficient secretion of type 5b–e proteins. A summary of AT secretion is found in Figure 16.4.
Structure of T5SS domains
The structure of many AT proteins has been solved, including EspP and Hbp from E. coli strains. These structures have revealed a domain architecture comprising a signal sequence, the passenger domain, an autochaperone domain, an α-helix linker followed by the translocation domain. The translocation domain of AT proteins is highly conserved and consists of β-pleated sheets in the form of a β-barrel akin to most other integral outer-membrane proteins (Loveless and Saier, 1997; Barnard et al., 2007; Tajima et al., 2010). Although diverse in sequence, all solved AT β-barrels contain 12 antiparallel strands connected by extracellular loops and periplasmic turns of varying length, with a narrow (~1 × 1.25 nm) hydrophilic pore. Translocation domains also share a consensus amino acid motif at the C-terminus (Struyve et al., 1991; Jose et al., 1995; Loveless and Saier, 1997). The C-terminal 9 aa are generally alternating aromatic/hydrophobic and charged/hydrophilic with the last residue a tryptophan or phenylalanine. This sequence (in particular the C-terminal three residues) is predicted to play a role in outer-membrane localization and/or stability of outer-membrane proteins.
Encoded just before the translocation domain, AT proteins have a single α-helix linker, found to reside within the barrel lumen. The length of the linker is determined by whether the passenger domain remains attached (long), is cleaved extracellularly (long) or cleaved within the barrel (short) (Barnard et al., 2007; Tajima et al., 2010). The α-helix also ensures the pore is blocked post-translocation preserving the integrity of the outer membrane. In addition to this α-helix, in the two solved E. coli ATs, a long extracellular loop of the translocation domain also folds into the barrel, closing the pore from the outside (Barnard et al., 2007).
Passenger domains of AT proteins are diverse in sequence and function and thus structures are different between proteins, however there are some commonalities. Above 97% of AT passenger domains are predicted to form a right-handed β-helical structure despite large diversity in sequence, length, and function. The right-handed β-helical structure was first solved for the T5SS protein, Pertactin (Emsley et al., 1994, 1996). β-Helical structures typically contain three β sheets separated by three turns giving the protein a ‘V’ shape in cross-section (Jenkins and Pickersgill, 2001). A complete turn of the β-helix is known as a coil, with different proteins having varying numbers of coils. The structure displays extensive ‘stacking’ across its coils, whereby similar aliphatic residues occupy equivalent positions in neighboring β sheets leading to ridges of aliphatic residues across the coils (Jenkins and Pickersgill, 2001). This right-handed β-helical structure is thought to be conserved in AT proteins because the passenger domain adopts a predominantly unfolded conformation during its passage through the outer membrane, a process that occurs independent of ATP and proton gradients (Junker et al., 2006). The conserved β-helical structure may contribute to protein folding after transport through the translocation domain and may also play a role in presenting an adhesive functional tip away from the cell surface (Klemm et al., 2004; Junker et al., 2006).
The β-helical structure of the passenger domain also allows functional groups to be inserted within turns that protrude from the β helix without disturbing its structural integrity (Emsley et al., 1996). These functional groups can be small, such as the tripeptide Arg-Gly-Asp (RGD) adhesion motif of the E. coli adhesin Antigen 43 (Fernandez and Weiss, 1994; Klemm et al., 2004) or large globular domains with various activities. The serine protease ATs of Enterobacteriacae (SPATEs) are the best examples of this globular structure. The crystal structures of three E. coli SPATE proteins, Hbp, EspP, and Tsh, have been solved with all including an extended right-handed β-helical structure that forms the spine and a globular subdomain reminiscent of the chymotrypsin family of serine proteases, which carries the proteolytic function of the passenger. In Hbp and Tsh there is also a second subdomain (termed domain 2) that adopts a chitinase b-like fold but for which a functional role has not yet been identified (Figure 16.5).
FIGURE 16.5 Crystal structures of the passenger domains of E. coli T5SS proteins. Hbp is a type 5a secreted SPATE with the typical stacked β-helices and globular domain. The β-barrel is composed of 12 strands with an α-helix linker. Intimin is a type 5e secreted protein with three Ig-like domains and a lectin-like domain which binds Tir. The β-barrel is composed of 12 strands with a periplasmic LysM domain. EibD is a type 5c secreted protein. Trimeric in structure it has clear head, stalk and neck regions. Figures adapted from protein databank (Otto et al., 1998; Luo et al., 2000; Tajima et al., 2010; Leo et al., 2011; Fairman et al., 2012).
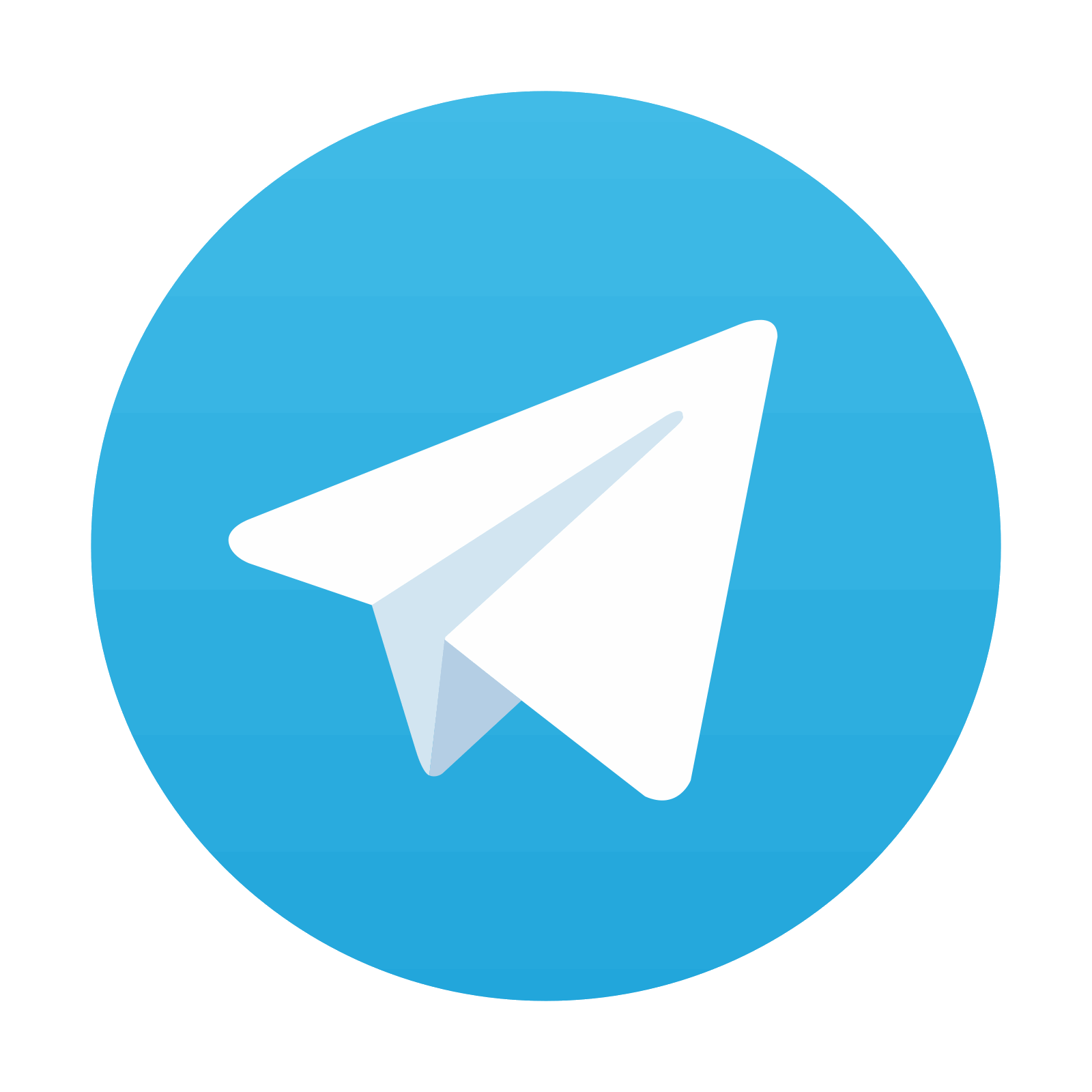
Stay updated, free articles. Join our Telegram channel
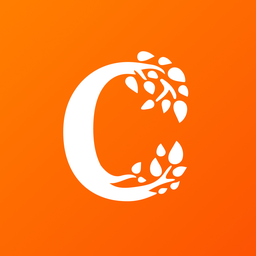
Full access? Get Clinical Tree
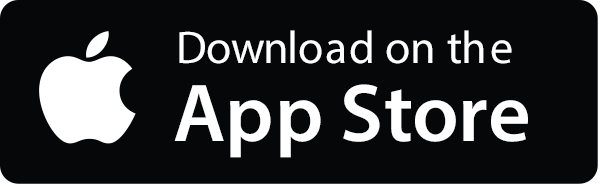
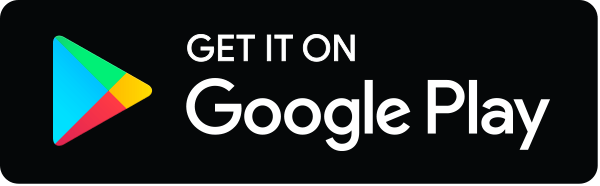