and Miguel A. R. B. Castanho2
(1)
Instituto de Bioquímica Médica Leopoldo de Meis, Federal University of Rio de Janeiro, Rio de Janeiro, Rio de Janeiro, Brazil
(2)
Institute of Biochemistry and Institute of Molecular Medicine, School of Medicine, University of Lisbon, Lisbon, Portugal
Carbohydrates, lipids , and proteins are the main components of foods and serve as “fuel” molecules that provide energy for the organism. A regular meal is generally composed of 45–65 % carbohydrates, 20–35 % lipids, and 10–30 % protein . After ingestion, these nutrients are broken down into smaller molecules, which are subsequently absorbed and metabolized (see Chap. 7).
The end products of carbohydrate and protein digestion are monosaccharides (mainly glucose ) and small peptides and amino acid s , respectively, which reach the portal vein after absorption by intestinal cells (Fig. 8.1). Before reaching the systemic circulation, these metabolites pass through the liver , which absorbs and stores from ½ to ¾ of the nutrients coming from the digestive tract (see Box 8.1).
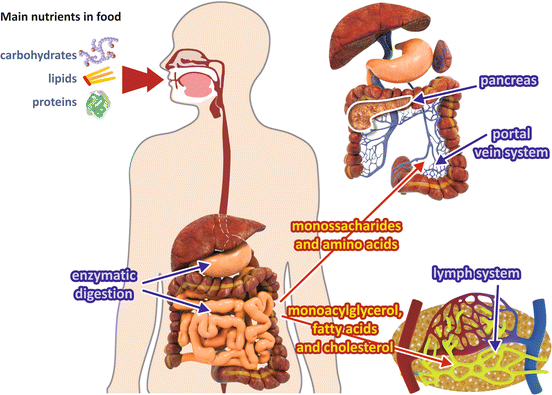
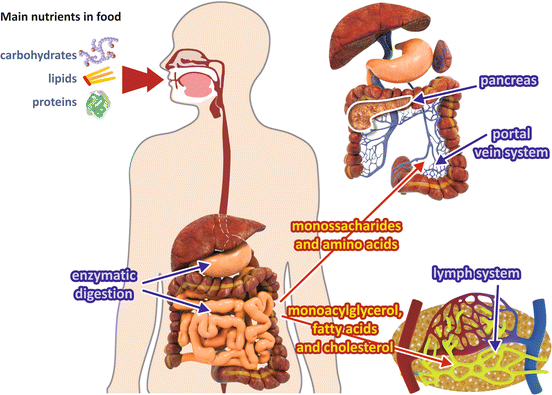
Fig. 8.1
Absorption of the main nutrients. Digestion of carbohydrates and proteins generates monosaccharides and amino acid s that are absorbed and travel through the portal vein system to the liver , entering the general circulation by the way of the hepatic vein. Digestion of lipids generates fatty acid s and monoacylglycerol, which are released into the lymph
Monoacylglycerol and long-chain fatty acid s which originated from lipid digestion are not soluble in aqueous medium and need specific transport mechanisms. They are reconverted to triacylglycerols in the intestinal mucosal cells and then are released into the lymph in the form of lipoproteins named chylomicrons (Fig. 8.1).
Box 8.1: The Hepatic Portal System
Blood is supplied to the liver by the hepatic portal vein and hepatic arteries. About 75 % of the blood entering the liver is venous blood drained from the intestine, pancreas , and spleen, which converges to the portal vein. Thus, everything absorbed by the digestive tract, including nutrients and toxins, as well as the pancreatic secretions and the blood cells and their degradation products released from the spleen, passes through the liver before reaching the systemic circulation. The remaining 25 % of the blood supply to the liver is arterial blood coming from the hepatic arteries.
The functional units of the liver are the hepatic lobules, a polygonal arrangement of plates of hepatocytes radiating outward a central vein (see figure). The hepatocytes make contact with the blood in the sinusoids, vascular channels that receive the blood coming from the terminal branches of both the portal vein and hepatic arteries. The sinusoids are lined with highly fenestrated endothelial cells, allowing a considerable amount of plasma to be filtered into the space between the endothelium and hepatocytes. Blood flows through the sinusoids and empties into the central vein of each lobule that coalesces into hepatic veins, which leave the liver. Of special importance in the context of this chapter is the fact that the liver is responsible for removing part of glucose from the blood, converting it into glycogen or lipids , before it reaches the systemic circulation.
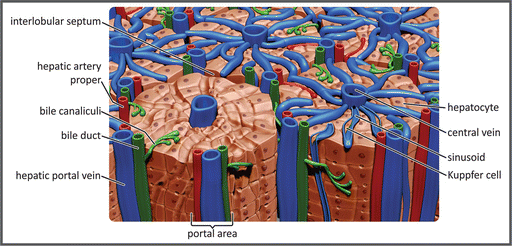
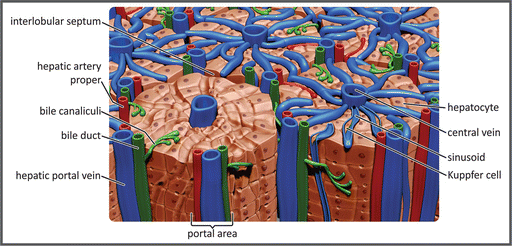
Schematic representation of the hepatic lobules. Portal vein branches and the sinusoids, carrying venous blood, are shown in blue, and the hepatic artery branches are shown in red. The bile ducts that transport the bile from the hepatocytes to the gallbladder or the duodenum are shown in green
This chapter will focus on the metabolic adaptations that take place after the ingestion of nutrients, which allow the components of the diet to be used as energy sources or to be stored as reserves that will be mobilized in the postabsorptive or fasting states. This main topic will be introduced with a discussion on how the cells can sense the variations in blood glucose concentration, how they respond to this, and how this sensing mechanism controls the secretion of insulin . Then, we will discuss in detail the metabolic pathway s involved in the biosynthesis of the main energy storage molecules, glycogen and triacylglycerol . Additionally, we will present the mechanism of action of insulin and its effects on different target tissues, giving an overview of the fate of the main metabolites absorbed after a meal in different cell types.
8.1 Glucose Sensing by Cells
The increase in blood glucose concentration is the main signal that transmits the information that food was ingested to most cells in human body, directly or indirectly by regulating the secretion of different hormones, which in turn control the energy metabolism . After a carbohydrate-rich meal, the increase in blood glucose concentration triggers the secretion of the hormone insulin (Fig. 8.2a). The action of this hormone on its target cells as well as the increase in glycemia itself produce a rapid glucose utilization by different tissues, both as energy source and as the precursor for the synthesis of storage molecules.
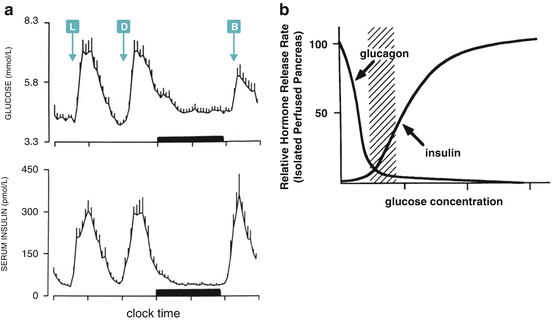
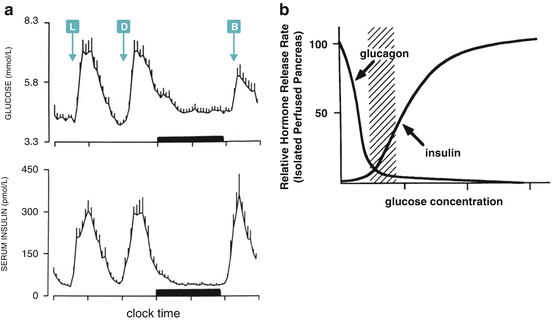
Fig. 8.2
(a) Day profile of plasma glucose and serum insulin of nine human subjects (mean + SE). L, D, and B indicate lunch, dinner, and breakfast time, respectively. The black bar represents the sleep period (Reproduced with permission from Biston et al. Hypertension 28:863–871, 1996). (b) Hormone secretion profile in isolated perfused pancreas as a function of glucose concentration. The dashed region corresponds to the physiological blood glucose range (Reproduced with permission of the American Society for Clinical Investigation, from Matschinsky et al. J. Clin. Invest. 92:2092–2098, 1993)
Glucose metabolism homeostasis depends on rapid responses to changes in glycemia. These responses occur when blood glucose concentration decreases (see Chap. 9), but also when glycemia rises. In both cases, the metabolic adaptations operate until the basal level of blood glucose concentration, which is approximately 5 mM, is reestablished (Fig. 8.2a). This set point is maintained due to the opposite effects of glucose on the rate of pancreatic secretion of the hormones glucagon and insulin , which shows a crossover point of 5 mM glucose (Fig. 8.2b), concentration below which glucagon action predominates, whereas above it insulin effects prevail.
Insulin is produced and secreted by the β-cells of pancreatic Langerhans islets. The mechanism involved in secretion induction will be discussed in Sect. 8.4, but a question that may be posed at this point is: How can the β-cells sense the increase in blood glucose concentration and promptly respond by increasing insulin secretion?
To answer this question, we will first analyze two important steps that precede and determine glucose utilization in cellular metabolism : (a) the transport of glucose across the plasma membrane and (b) its phosphorylation to glucose-6-phosphate .
Glucose enters the cells by facilitated diffusion mediated by membrane transport protein s , the glucose transporters (GLUTs). Fourteen GLUT isoforms are expressed in human cells, which are characterized by distinct kinetic and/or regulatory properties, substrate specificity, cellular location, as well as tissue-specific expression. The best studied GLUTs are the isoforms 1–4 (Table 8.1), which play a central role in glucose homeostasis by conferring specific properties to glucose uptake depending on the cell type. The other isoforms seem to transport fructose , myoinositol and urate, in addition to glucose, and are probably also involved in the transport substrates yet to be identified.
Table 8.1
Properties of the four best studied GLUT isoforms
Transporter | K M (mM) | Distribution | Features | Putative Structurea |
---|---|---|---|---|
GLUT-1 | 1–2 | Ubiqitous, erythrocytes | Constitutive glucose transporter | ![]() |
GLUT-2 | 20 | Liver, β-cells, intestine, kidney | Low affinity, high capacity transporter | |
GLUT-3 | 1 | Neurons, Placenta | Low affinity transporter | |
GLUT-4 | 5 | Adipose tissue, skeletal muscle heart | Insulin-dependent transporter |
In the liver and pancreatic β-cells, the uptake of glucose occurs predominantly through GLUT2, which is characterized by a very high K M for glucose uptake (~17 mM) and by a very high expression level that gives these cells a high capacity for transport. These properties ensure the fast equalization of extracellular and cytosolic concentrations of glucose after any change in the physiological glycemic levels.
Thus, although GLUT proteins are important for the regulation of the glucose uptake in other tissues (see Sect. 8.4.3 for glucose transport in the muscle and adipose tissue), in the case of β-cells GLUT2 properties are important for balancing the extra- and intracellular glucose concentrations.
To complement the answer to our question about how β-cells sense the changes in glycemia, we have also to take into account the next step of glucose utilization by cells. Glucose metabolization starts with its phosphorylation to glucose-6-phosphate , a reaction catalyzed by a family of enzymes named hexokinases (HK). Once glucose is phosphorylated, it cannot leave the cell unless the phosphate group is removed by the action of glucose-6-phosphatase . Since this enzyme is only expressed in liver and kidney cells (see Sect. 9.3.1), glucose phosphorylation in most cells, including β-cells, marks it for metabolization inside the cell.
There are four isoforms of HKs (HK 1–4). The isoform expressed in β-cells is the HK 4, also named glucokinase (GK) since glucose is its preferred substrate. GK is a monomer with low affinity for the glucose, with an S 0.5 of 8 mM, and cooperativeness with a Hill number of 1.7, giving to the curve that describes its activity as a function of glucose concentration an inflection point of approximately 4 mM glucose (Fig. 8.3 ). Additionally, unlike other HK isoforms, GK is not inhibited by the reaction product, glucose-6-phosphate . These kinetic properties make it possible that changes in GK activity occur exactly over the range of physiological blood glucose concentrations, as can easily be seen in Fig. 8.3 , which compares the activity profile of HK isoforms as a function of glucose concentration. HK 1 activity is the same (and maximal) over all glucose concentrations in the physiological range, while a great increment in glucose phosphorylation by GK occurs as the glucose concentration increases from 2 to 12 mM. Reminding that extra- and intracellular concentrations of glucose are equalized due to the properties of GLUT2 in β-cells, the kinetic features of GK makes it possible that the rate of glucose metabolization in β-cells is determined by blood glucose concentration, making GK the intracellular sensor of glycemia. Nevertheless, mutations in the gene encoding GK cause different pathologies characterized by the impairment in glycemia control (Box 8.2).
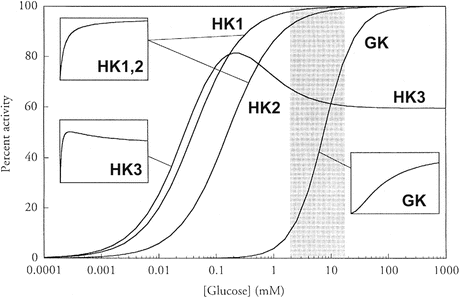
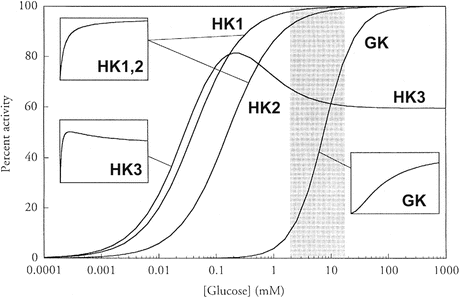
Fig. 8.3
Comparison of the kinetic properties of HK isoforms. The physiological range of blood glucose concentration is indicated by the gray section. The insets show the curves redrawn with a linear scale of glucose concentration to better illustrate the kinetic profile (Reproduced from Cardenas et al. Biochim. Biophys. Acta 1401:242–264, 1998, with permission from Elsevier)
GK seems to play the role of glucose sensor in all GK-containing cells, which include, besides the β-cells, the hepatocytes , the specialized hypothalamic neurons , and the endocrine enterocytes. However, the responses to glucose sensing may be different in each of these cells. In β-cells, the increase in blood glucose concentration stimulates insulin secretion (see Sect. 8.4.2), whereas in hepatic cells, it induces the expression of glycolytic and lipogenic genes. Additionally, glucose sensing in hypothalamic cells participates in the regulation of food ingestion and energy expenditure, impacting on the control of body weight, as it will be discussed in Chap. 11.
Box 8.2: GK-Related Pathologies
The role of GK as the glucose sensor in β-cells is strongly supported by the consequences of several mutations in GK gene, which are manifested in at least three distinct pathologies: (a) persistent hyperinsulinemic hypoglycemia (PHHI), caused by mutations that activate the enzyme ; (b) permanent neonatal diabetes mellitus (PNDM), which occurs in newborns carrying inactivating mutations in the two alleles of the gene; and (c) maturity-onset diabetes of the young (MODY), occurring due to one defective allele with a mutation that inactivates the enzyme. The β-cell threshold for glucose stimulation of insulin release (GSIR) may be as low as 1.5 mM in PHHI patients because of lowered glucose S 0.5 and/or increase in k cat, while it may be increased to 7 mM due to a single inactivating mutation in a MODY patient (figure). Therefore, these syndromes can be explained on the basis that glucose concentration threshold for GSIR is determined by the catalytic capacity and/or in the substrate affinity of β-cell GK, supporting the concept that this enzyme plays a role in glucose sensing.
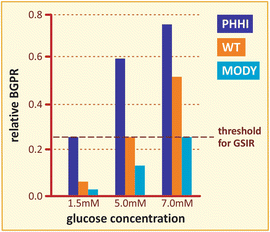
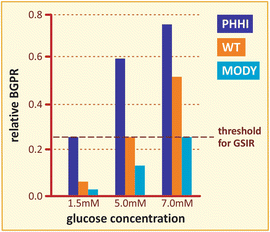
Comparison of the relative glucose phosphorylation rate as a function of glucose concentration for the wild type and two different mutants of GK. The dashed line indicates the threshold for glucose stimulation of insulin release, GSIR (Based on data from Matschinsky. Diabetes 51:S394–S404, 2002)
Before describing the mechanisms involved in insulin secretion and the direct effects of this hormone on the regulation of metabolism of different target cells, we will present in detail the main metabolic pathway s that are activated when glycemia rises.
Figure 8.2a shows how glucose is rapidly removed from the bloodstream in a period of 2 h after a carbohydrate-rich meal. In this period, the major fates of glucose are (a) its use as energy source by most, if not all, of the cells in the body (which does not imply that it is the only energy source of the human body); (b) its incorporation into glycogen molecules, in which glucose is stored by polymerization, therefore, without major changes in its molecular structure; and (c) its transformation into fatty acid s , which are incorporated in the triacylglycerol molecules, the main energy storage molecules in the human body (Fig. 8.4).
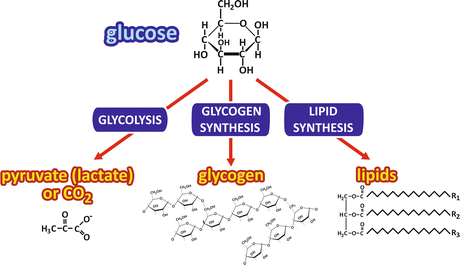
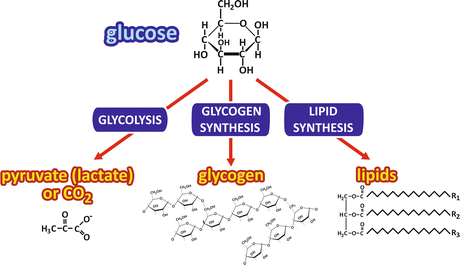
Fig. 8.4
Possible fates of glucose after its uptake by the cells. The metabolic pathway s that may be followed by glucose are shown in the blue boxes
The use of glucose as energy source has already been discussed in Chaps. 6 and 7. In this chapter, we will detail the metabolic pathway s that convert glucose in energy reserves, the synthesis of glycogen and the synthesis of lipids . We will also present the pentose-phosphate pathway, an additional pathway for glucose-6-phosphate oxidation that generates NADPH for the reductive biosynthesis and pentose phosphate for nucleotide synthesis.
8.2 Biosynthesis of Glycogen
Glycogen is a branched polymer of glucose that forms granular structures corresponding to aggregates of glycogen itself associated to the enzymes involved in its synthesis and degradation as well as their regulatory machinery (Fig. 8.5).
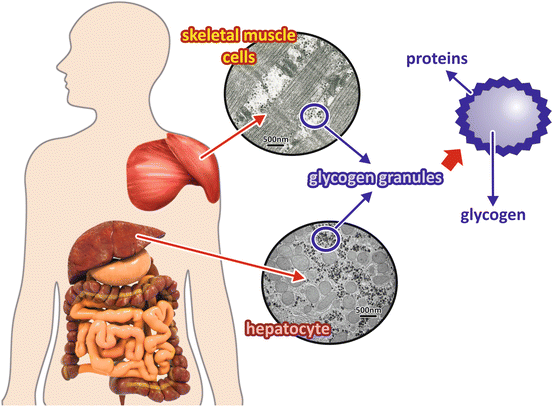
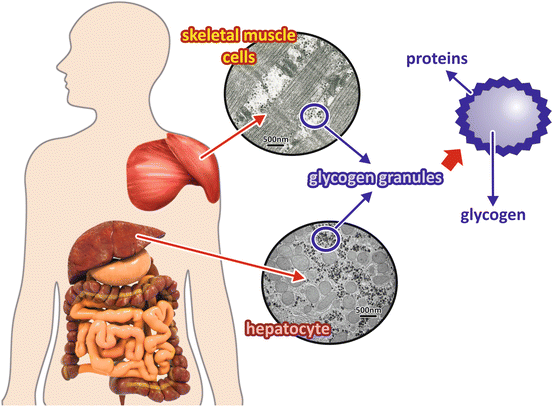
Fig. 8.5
Liver and muscle glycogen granules. Glycogen, a polymer of glucose molecules, is stored mainly in the liver and in muscles as granules with the glycogen molecule in the center surrounded by proteins and enzymes involved in its metabolism . hepatocyte The electron micrographs show sections of a skeletal muscle cell (top) and a hepatocyte (bottom) in which glycogen depots can be seen as electron dense. (Transmission electron micrograph images: skeletal muscle cell section, reprinted from the archives of Prof. David Ferreira, with the permission of Karin David Ferreira; hepatocyte section, courtesy from Prof. Marlene Benchimol)
In humans, glycogen is accumulated mainly in the liver and skeletal muscle in response to an increase in blood glucose concentration that generally occurs after a meal. It serves as an immediate source of glucose, which in the liver is released to maintain glycemia (see Sect. 9.2.1), while in muscle it serves as a fuel reserve to ensure ATP synthesis during intense contractile activity (see Sect. 10.4.2). The general pathways for storing and mobilizing glycogen are the same in both tissues, with slight differences in the enzymes , especially with respect to the mechanisms of their regulation.
In the beginning of the twentieth century, Carl and Gerty Cori discovered and studied glycogen phosphorylase (GP), the enzyme that catalyzes glycogen degradation (see Sect. 9.2.1). Since they demonstrated using different tissue preparations that the reaction of catalyzed by this enzyme was reversible in vitro, the synthesis of glycogen was firstly believed to occur as the reversal of glycogenolysis.
The existence of a different pathway for glycogen synthesis became more evident after the discovery of the cause of McArdle’s disease, the most common disorder of glycogen metabolism , which is caused by a mutation in the muscle isoform of GP, resulting in a nonfunctioning enzyme and in a complete block of glycogen degradation in muscle. Patients with McArdle’s disease have chronic high muscle glycogen levels, showing that glycogen synthesis occurs independently of GP activity.
Both in the liver and muscle , blood glucose is the major precursor for glycogen synthesis , which is mainly regulated by insulin action, as it will be discussed in Sect. 8.4. In the skeletal muscle, the transport of glucose into the cells is also insulin dependent (GLUT4 transporter).
The synthesis of glycogen can be divided in two distinct parts, the initiation and the elongation phases. The initiation phase is required when a new glycogen molecule is synthesized and is mediated by glycogenin , a protein that, besides catalyzing the incorporation of the first glucose units in the nascent glycogen chain, remains covalently linked to the final glycogen molecule. The elongation phase depends on two different enzymes : (a) the glycogen synthase (GS), which catalyzes the successive addition of α-1,4-linked glucose units to a nonreducing end of the glycogen branch, and (b) the branching enzyme , which has a glucosyl(4 → 6)transferase activity that creates the α-1,6-glucosidic bond that starts a new branch.
For both initiation and elongation reactions, the donor of the glucose units to be incorporated in the glycogen molecule is UDP-glucose .
The role of UDP-glucose as a donor of glucose units for the synthesis of disaccharides and glycogen was discovered by Luis Leloir, an Argentine biochemist. After Leloir’s findings, it became clear that the formation of a sugar nucleotide was a general activation step in the reactions of hexose polymerization, including the formation of disaccharides, glycogen, extracellular polysaccharides , and aminohexoses and deoxyhexoses found in some of these polysaccharides. Due to this great scientific contribution that opened the way for the understanding of carbohydrate biosynthesis, Leloir was awarded the Nobel Prize in Chemistry in 1970.
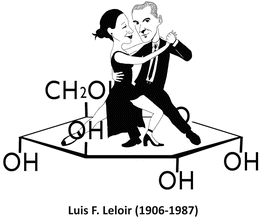
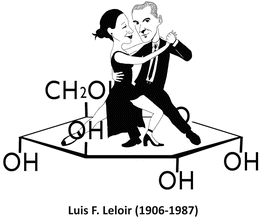
8.2.1 Formation of UDP-Glucose
The pathway from blood glucose to UDP-glucose differs in the liver and muscle in the beginning but converges to the same reactions in the end (Fig. 8.6a).
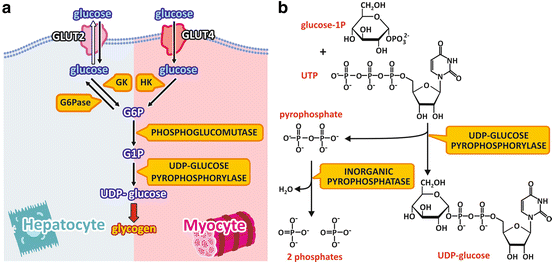
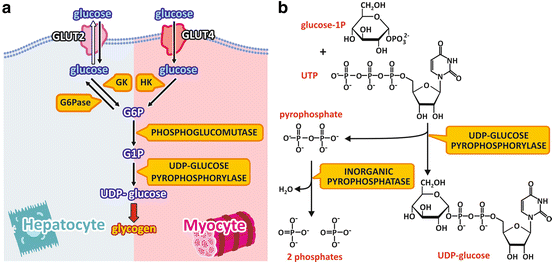
Fig. 8.6
(a) Pathway from blood glucose to UDP-glucose in the liver and muscle cells. Glucose enters the cell through GLUT2 or GLUT4 and is phosphorylated by GK or HK in the liver or muscle cells, respectively. Glucose-6-phosphate (G6P) is converted to glucose-1-phosphate (G1P), which is the substrate to the formation of UDP-glucose in both cell types. UDP-glucose is the substrate for glycogen synthesis . The names of the enzymes are highlighted in yellow boxes. (b) Reaction for UDP-glucose synthesis catalyzed by UDP-glucose pyrophosphorylase
In the liver cells, glucose enters the cells through GLUT2 and is phosphorylated by GK. As already discussed for β-cells (see Sect. 8.1), this makes the synthesis of glucose-6-phosphate dependent on glucose concentration in the blood. Additionally, since the liver cells express the enzyme glucose-6-phosphatase , which removes the phosphate group from glucose-6-phosphate, when intracellular concentration of glucose-6-phosphate becomes high, some of the molecules may be dephosphorylated to glucose, which then leaves the cells. Thus, part of glucose molecules that enter the liver are metabolized in this organ, but the excess returns to the bloodstream to be used by other tissues.
In the muscle cells, the GLUT4 transports glucose into the cytoplasm. This transport is dependent on insulin action, as it will be discussed in detail in Sect. 8.4. Once inside the cell, glucose is phosphorylated to glucose-6-phosphate by HK, which has a high affinity for glucose.
Once glucose -6-phosphate is formed, in both the liver and muscle , the enzyme phosphoglucomutase converts glucose-6-phosphate to glucose-1-phosphate , which is the substrate to UDP-glucose synthesis. Glucose-1-phosphate reacts with UTP to form UDP-glucose, which is the immediate precursor for glycogen synthesis (Fig. 8.6b). This reaction is catalyzed by UDP-glucose pyrophosphorylase, with the formation of pyrophosphate besides UDP-glucose. Although the enzyme name, UDP-glucose pyrophosphorylase, refers to the reverse reaction, the synthesis of UDP-glucose is irreversible in physiological conditions. This occurs because pyrophosphate is readily hydrolyzed by the enzyme inorganic phosphatase, in a very exergonic reaction that pushes the glycogen synthesis forward.
8.2.2 Reactions for the Initiation of Glycogen Synthesis from UDP-Glucose
The initiation of glycogen synthesis depends on a very unusual protein , named glycogenin (Fig. 8.7). This protein occupies the core of glycogen molecule and is also the enzyme that catalyzes the initiation of glycogen synthesis.
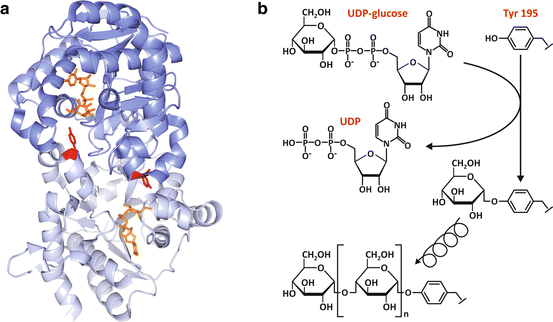
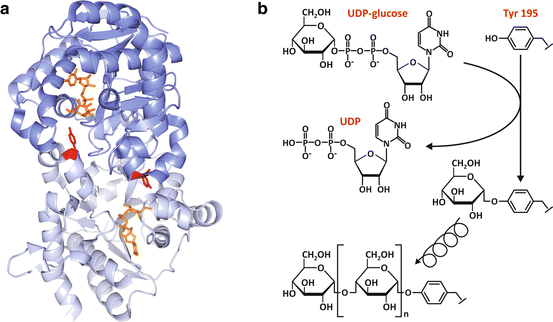
Fig. 8.7
(a) Structure of dimeric human glycogenin -1 (PDB 3T7O), highlighting Tyr195 (red), the residue that is glycosylated by the transfer of a glucose unit from UDP-glucose (orange), which is shown bound to the nucleotide -binding domain. (b) The two chemically distinct reactions that are catalyzed by glycogenin: the initial glucosylation of Tyr195 through the formation of a glucose-O-tyrosyl linkage (top) and the subsequent formation of the α-1,4-glucosidic linkages (bottom)
Glycogenin is a member of glucosyltransferase superfamily. It is dimeric and shares with other glucosyltransferases the nucleotide -binding fold comprising a four-stranded αβ-domain that is responsible for the majority of the interactions with the UDP moiety of UDP-glucose (Fig. 8.7a). In mammals, there are two tissue-specific isoforms: glycogenin -1 predominantly expressed in muscle and glycogenin-2 mainly expressed in the liver .
The initial reaction catalyzed by glycogenin is unique, since the protein is the substrate, the catalyst, and the product of the reaction. First, glycogenin catalyzes the glucosylation of its Tyr195 by transferring a glucose unit from UDP-glucose to form a glucose-1-O-tyrosyl linkage (Fig. 8.7b). Then, glycogenin sequentially incorporates α-1,4-linked glucose residues to the nascent glycogen chain starting from the Tyr195-linked glucose and using UDP-glucose as substrate until the chain reaches 8 glucose residues, with the chain remaining attached to glycogenin (Fig. 8.7b).
8.2.3 Reactions for the Elongation of Glycogen Chain
Elongation of glycogen chain depends on two different enzymes , the GS and the branching enzyme .
GS catalyzes the formation of an α-1,4-glucosidic bond between a glucose unit from UDP-glucose and a nonreducing end of a glycogen chain (Fig. 8.8a).
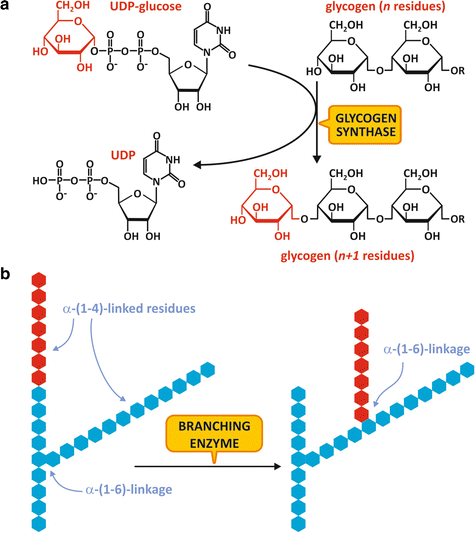
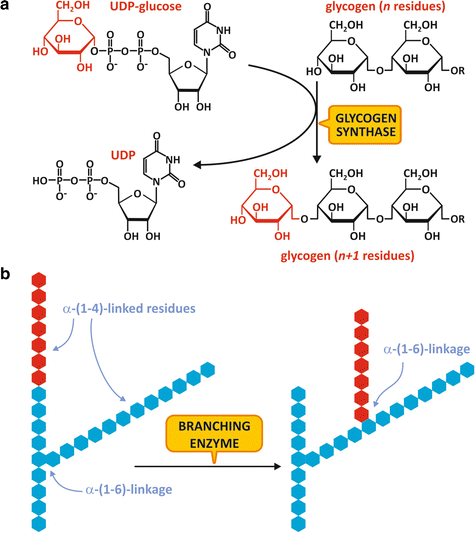
Fig. 8.8
(a) Reaction for glycogen chain elongation, catalyzed by GS. (b) Reaction for glycogen ramification, catalyzed by the branching enzyme
When the glycogen chain reaches at least 11 residues, it becomes a substrate for the branching enzyme , which catalyzes the transfer of a terminal segment of 7 glucose residues to a hydroxyl group on the position 6 of a glucose residue in the same or in a neighboring chain, creating an α-1,6-linked branch (Fig. 8.8b). Extra glucose residues may be added to the new branch or to the original chain by the action of GS.
Branching enzyme activity is important not only because the branches increase glycogen solubility inside the cell but also because it increases the number of nonreducing ends that are the sites of action of GS and GP, making glycogen molecule more readily metabolized.
Glycogen molecule usually grows until 12 tiers are formed, each of them with 12–14 glucose residues and two branches, resulting in approximately 55,000 glucose residues incorporated, which gives to the molecule an average molecular weight of 107 and a diameter of 21 nm.
8.2.4 Regulation of Glycogen Synthesis
GS is the key enzyme in the regulation of glycogen synthesis . GS activity is inhibited by phosphorylation , which can occur in multiple sites by at least 11 different protein kinase s (Box 8.3).
Box 8.3: The Multisite Phosphorylation in GS Inactivation
In humans, there are two isoforms of GS with 70 % identity: the muscle isoform, which is expressed in most tissues, and the liver isoform, whose expression is tissue specific. The catalytic site of the enzyme is located at the central region of its primary sequence and presents the highest degree of identity between the two isoforms (80 %). The N- and C-terminal ends show lower degree of homology between the isoforms (50 and 46 %, respectively) and contain multiple phosphorylation sites: 2 and 2a in the N-terminus and 3a, 3b, 3c, 4, 5, 1a, and 1b in the C-terminus (see figure). At least 11 different protein kinase s are involved in GS phosphorylation, which results in enzyme inactivation: cAMP -dependent protein kinase (PKA ), Ca2+-calmodulin protein kinase (CaMK), protein kinase C (PKC ), phosphorylase kinase (PK), cGMP-dependent protein kinase (PKG), AMP -activated protein kinase (AMPK), ribosomal protein S6 protein kinase II (S6KII), mitogen-associated protein kinase 2 (MAPK2), casein kinase I (CKI), glycogen synthase kinase 3 (GSK3), and casein kinase II (CKII). It is interesting to note that this mechanism of GS regulation involving multiple phosphorylations is very different from that of GP, the enzyme that catalyzes glycogen breakdown, which is activated by phosphorylation on a single serine residue by phosphorylase kinase (see Sect. 9.2.2).
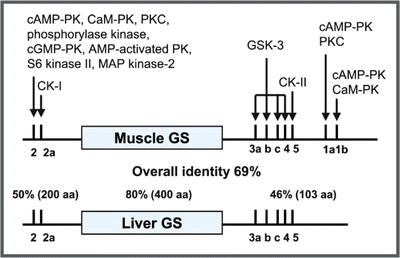
Schematic representation of the primary sequence of the muscle and liver GS isoforms showing the phosphorylation sites and the possible protein kinase s involved in phosphorylation. (Reproduced from Ferrer et al. FEBS Lett. 546:127–132, 2003, with permission from Elsevier)
Although phosphorylation causes GS inhibition, this effect can be completely overcome by the binding of glucose -6-phosphate , the major allosteric activator of GS (Fig. 8.9).
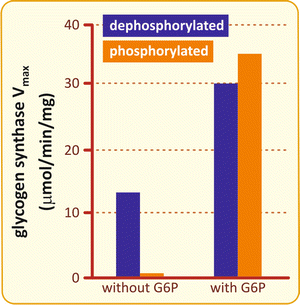
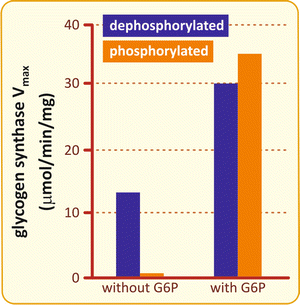
Fig. 8.9
Effects of phosphorylation and glucose -6-phosphate on GS activity. GS is activated by glucose-6-phosphate (G6P) either when it is phosphorylated (orange) or dephosphorylated (blue), but without G6P, the basal activity is inhibited by phosphorylation (Based on data from Pederson et al. J. Biol. Chem. 275:27753–27761, 2000)
The molecular mechanisms that underlie the switch between the inhibitory effect of phosphorylation and the enzyme activation by glucose -6-phosphate depend on a cluster of six conserved Arg residues located in the C-terminal region of the enzyme (Box 8.4).
Box 8.4: The Three-State Model for GS Regulation
The crystal structure of yeast GS revealed that the eukaryotic enzymes exist as tetramers. The location of the helix containing the conserved Arg residues indicates that these residues interact with the phosphate groups of either glucose-6-phosphate or the phosphorylated sites, suggesting a three-state model for the regulation of GS activity (see figure). The I state, which corresponds to the dephosphorylated form, shows an intermediate basal activity due to its low affinity for glycogen . The T state is the phosphorylated form of the enzyme , in which the interaction between the phosphorylated residues and the conserved Arg locks the active site cleft, leading to enzyme inactivation. The R state is achieved by the binding of glucose-6-phosphate, whose phosphate group interacts with two conserved Arg residues, resulting in the opening of the active site cleft, allowing UDP-glucose and glycogen to bind and react.
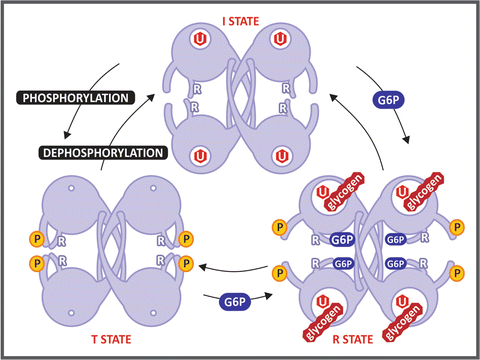
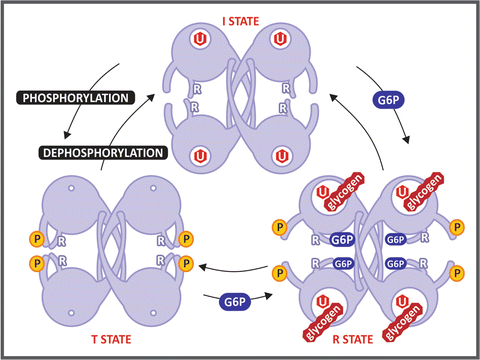
Schematic representation of GS conformational states proposed in Baskaran et al. Proc Natl Acad Sci USA 107:17563–17568, 2010. The regulatory Arg residues (labeled with an R) interact with the phosphate groups of Tyr 668 in the phosphorylated form, locking the enzyme in the T state. Glucose-6-phosphate (G6P) binding frees this constraint, leading the enzyme to the R state, which is fully active. The hexagon labeled with U represents the UDP-glucose molecule
The major protein kinase involved in GS inactivation is the glycogen synthase kinase 3 (GSK3), which catalyzes the sequential incorporation of phosphate groups in adjacent phosphorylation sites of the enzyme . However, evidence accumulated during the 1980s indicated that GSK3 alone cannot phosphorylate and inactivate GS, being necessary a concerted action of more than one protein kinase (Box 8.5).
Box 8.5: Inactivation of GS by GSK3
The results of in vitro phosphorylation experiments, performed with the recombinant muscle GS, clearly demonstrated that GSK3 alone cannot phosphorylate and inactivate GS, requiring previous phosphorylation by the casein kinase II (see figure). Casein kinase II is able to incorporate one phosphate group (at the phosphorylation site 5; see Box 8.3) per subunit of GS, but this phosphorylation alone has little effect on the enzyme activity. On the other hand, once phosphorylated by casein kinase II, GS becomes an effective substrate for GSK3, which introduces additional four phosphate groups in the enzyme (at the phosphorylation sites 4, 3c, 3b, and 3a), resulting in a great decrease of its activity. This occurs because GSK3 needs to bind to a priming phosphate in order to catalyze the phosphorylation of the subsequent phosphorylation site (see the schematic representation in the figure of GSK3 structure). This latter site, when phosphorylated, becomes another site for GSK3 to bind and to act on the next available site. This sequential phosphorylation continues until five phosphate groups are incorporated in the GS C-terminal phosphorylation sites.
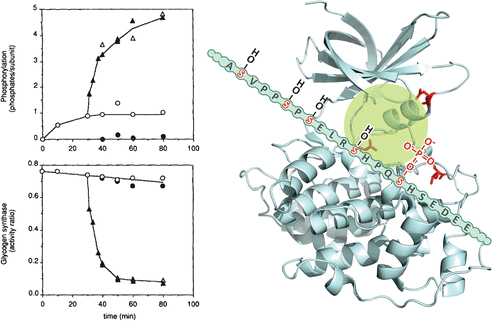
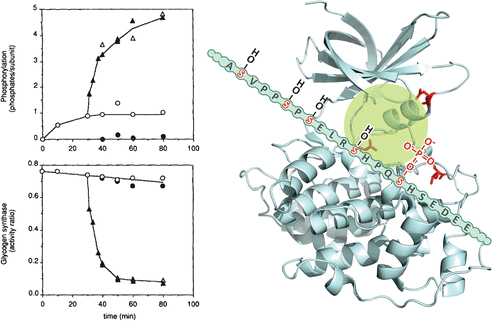
Left: quantification of phosphate groups incorporated (upper panel) and the effect on enzyme activity (lower panel) after incubation with casein kinase II alone (open circles), GSK3 alone (filled circles), or GSK3 added after 30 min incubation with casein kinase II (triangles) (Reproduced from Zhang et al. Arch. Biochem. Biophys. 304:219–225, 1993, with permission from Elsevier). Right: GSK3 structure (PDB 1H8F). The positively charged residues Arg96, Arg180, and Lys 205 (lateral chains shown in red) interact with the phosphate group incorporated in GS by casein kinase II, allowing the occupation of GSK3 active site (highlighted in green) with the GS subsequent phosphorylation . Thus, GS slides allowing the incorporated phosphate to interact with the positively charged GSK3 residues, allowing the next phosphorylation site to occupy the active site
It is interesting to point out that although GSK3 was firstly discovered as acting in insulin -mediated activation of GS, it is now recognized as a constitutively active protein kinase that phosphorylates a wide range of substrates. GSK3 is involved in many cellular processes, such as cell proliferation, neuronal function, oncogenesis, and embryonic development, besides glycogen metabolism and insulin signaling . Interestingly, many of GSK3 substrates, besides GS, need this “priming phosphorylation ” in a Ser/Thr residue located +4 residues of the site of GSK3 phosphorylation.
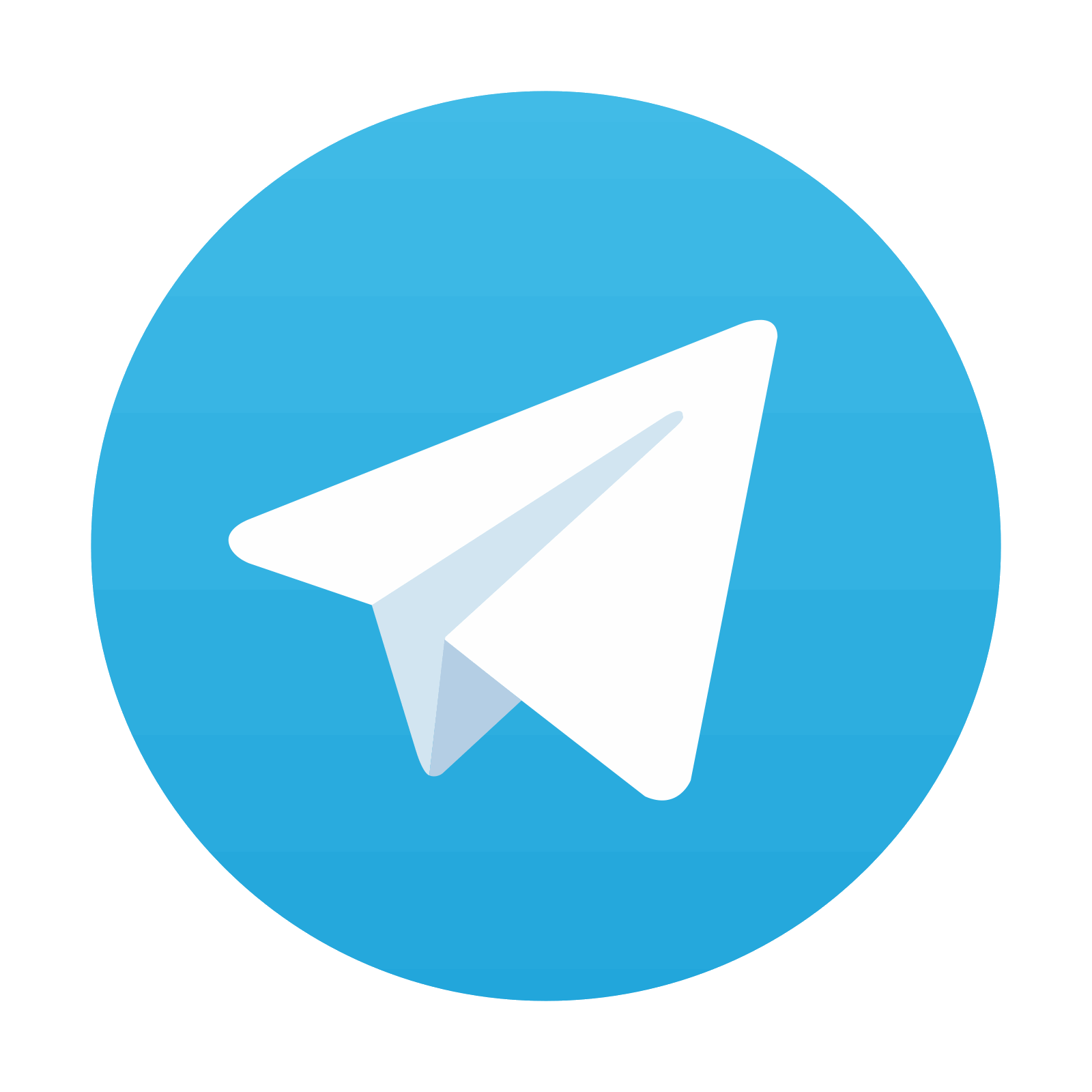
Stay updated, free articles. Join our Telegram channel
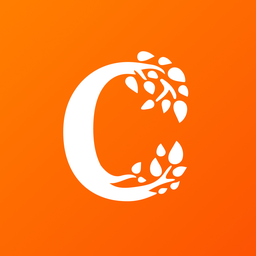
Full access? Get Clinical Tree
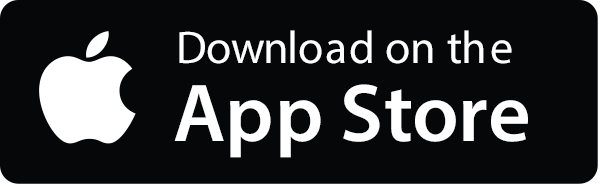
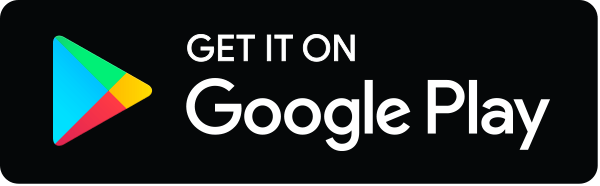
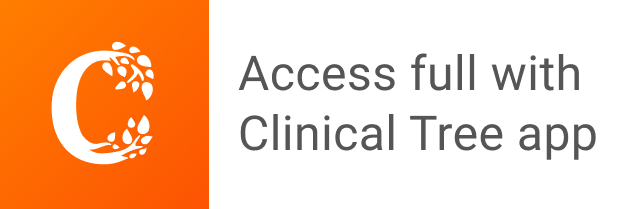