inactivation of tumour suppressor genes or changes in microRNA genes (encoding RNA molecules which regulate gene expression) will promote unregulated cell proliferation,


Activation of telomerase may also be important. Telomeres are found at the ends of chromosomes and their progressive shortening as healthy cells divide ultimately arrests further division. In cancer cells, telomerase activation extends the telomeres and promotes cell growth and division. Defective function of the tumour suppressor protein p53 may be a factor in reducing apoptosis and permitting cell proliferation. Growth factors secreted by cancer cells promote angiogenesis and increase blood supply to the tumour. Other secreted factors can impede the host’s immune response to the cancer cells. Genetic changes in cancer cells can give rise to metabolic changes or expression of transporters such as P-glycoprotein that confer resistance to chemotherapy (Ch. 2).
Antineoplastic drugs
The majority of antineoplastic drugs act on the process of DNA synthesis within the cancer cell, as summarised in Figure 52.1. Selectivity of these drugs for cancer cells compared with normal tissues is determined by the rate of DNA synthesis and cell division. Resting cells in the G0 phase (Fig. 52.2) are resistant to many antineoplastic drugs. Cell cycle-specific antineoplastic drugs, such as the antimetabolites (Fig. 52.2), work effectively only when the cells are in the appropriate phase of the cell cycle at the time of treatment. Non-cell cycle-specific antineoplastic drugs, such as the alkylating drugs, nitrosoureas and cisplatin, have a ‘hit-and-run’ action on DNA, and it is not critical when the cell is exposed because the drug effect becomes apparent when the cells attempt to divide.
The sensitivity of a cancer to treatment depends on its growth fraction, which is the fraction of cells undergoing mitosis at any time. For example, in Burkitt’s lymphoma almost 100% of neoplastic cells are undergoing division simultaneously and are they are very sensitive to chemotherapy, showing a dramatic response to a single dose of cyclophosphamide. In contrast, the growth fraction in a carcinoma of the colon is less than 5% of cells, resulting in its relative resistance to chemotherapy. However, metastases from colonic carcinoma deposited in the liver and elsewhere initially have a high growth fraction and are more sensitive to anti-cancer drugs.
Using in vitro cancer cell lines, it has been shown that:

In vivo, the immune system probably contributes to the final removal of residual malignant cells; however, most antineoplastic drugs compromise immunoresponsiveness, which will reduce this removal process. The periodicity of doses is probably less critical in vivo because cancer cell cycles are not synchronised within the target cell population between treatments. In clinical practice, dose intervals are often established to allow recovery of healthy cells from toxic effects of the treatment. Therefore, while these concepts apply to in vivo cancer treatment, risk–benefit considerations may change with successive treatments and preclude complete eradication of the tumour.
Resistance
Resistance to chemotherapeutic drugs may develop in a number of ways. These are explained later in the text for individual drugs, but include:






Unwanted effects
Cytotoxic antineoplastic drugs are among the most toxic compounds given to humans. Many have a therapeutic index of approximately 1, as the therapeutic dose is essentially the same as the toxic dose. Because drug action is usually greater in tissues with a high growth fraction, a number of normal, rapidly dividing non-malignant tissues are also affected. In addition to effects that occur in all rapidly dividing tissues, many chemotherapeutic drugs also have specific toxic effects on other tissues. Dosage regimens are usually designed so that normal tissues, especially bone marrow and gut, can recover between doses (Fig. 52.3).
Fig. 52.3 Hypothetical dosing schedule of anti-cancer drugs to allow recovery of normal tissues.
At least 109 tumour cells are usually present when tumours are first detectable. The malignant cells show a greater proportional kill than normal cells because a larger fraction is in division at any time. Theoretically, the response of the malignant cells to dose 2 would be greater than for dose 1 if cell cycles became synchronised and dose 2 was given during the correct phase of the growth cycle. A typical dose interval is 3–4 weeks.
Gastrointestinal tract
Mucosal cells have a rapid turnover. Toxicity can produce anorexia, mucosal ulceration or diarrhoea. A sore mouth is most common with fluorouracil, methotrexate and the anthracyclines. Nausea and vomiting are common, especially with alkylating drugs and cisplatin, and this may limit an individual’s ability to tolerate an optimal dosage regimen (see Ch. 32).
Bone marrow
Myelosuppression is a serious consequence of treatment and can lead to severe neutropenia, thrombocytopenia and sometimes anaemia. It often occurs 7–10 days after a cycle of chemotherapy, but is delayed with drugs such as melphalan and lomustine. These haematological consequences may limit the drug dosage that the person is able to tolerate. There is a high risk of both infection (neutropenic sepsis) and haemorrhage following cytotoxic chemotherapy.
Reproductive organs
Both sexes are affected and sterility can result, particularly after therapy with cyclophosphamide or cytarabine. Because of the mechanisms of action of cytotoxic drugs, most have teratogenic activity. Pregnant women should not be exposed to cytotoxic drugs for treatment or as members of the healthcare team. Alkylating agents or procarbazine can cause permanent male infertility. Drugs that mimic or affect the activity of sex hormones are frequently used for the treatment of breast or prostate cancer, and these inevitably produce adverse effects on sexual function.
Growing tissues in children
Of particular concern in children is the possibility that intensive cytotoxic chemotherapy can impair growth. Children treated with cytotoxic drugs for malignancy also have an increased risk of the subsequent development of a second malignancy (about 10%), which is often leukaemia.
Extravasation of intravenous drug
If anti-cancer drugs leak from a vein into the surrounding tissues, they can cause severe local tissue necrosis.
Tumour lysis syndrome
Rapid breakdown of malignant cells can produce hyperuricaemia, hyperkalaemia, hypophosphataemia, hypocalcaemia with consequent renal damage or arrhythmias. The syndrome is most common with treatment of non-Hodgkin’s lymphoma, Burkitt’s lymphoma and acute leukaemias. Tumour lysis syndrome can be ameliorated by good hydration and the prophylactic use of allopurinol (Ch. 31).
Drug combinations
It is common practice to treat many cancers with a combination of different antineoplastic drugs simultaneously. Potential combinations of drugs are investigated using in vitro and in vivo experiments before they are subjected to clinical evaluation in humans. The most successful combinations are those that show synergism in their actions on cancer cells, rather than a simple additive effect, while showing no increase in their systemic toxicity. Criteria for selecting ideal combinations are:


Specific antineoplastic drugs
The drug compendium at the end of this chapter outlines the licensed uses of individual drugs and notes any unusual or limiting toxicity.
Drugs affecting nucleic acid function
Mechanism of action and uses: The nitrogen mustards were developed from the sulphur mustard gases used as chemical warfare agents in World War I. These gases caused bone marrow suppression in addition to the respiratory toxicity for which they were developed. Replacement of the divalent sulphur atom by trivalent nitrogen allowed the introduction of a complex side chain, which resulted in a range of more stable nonvolatile drugs that could be given therapeutically under controlled conditions. Alkylating drugs contain side chains which undergo a metabolic activation step that involves loss of part of the molecule (for example the Cl is lost from –CH2CH2Cl) and yields a highly reactive product which binds to DNA or proteins. Many alkylating drugs are bifunctional (i.e. have two reactive groups). The reactive alkylating group(s) in the molecule may be:

The mechanism of action is by covalent binding to DNA (nitrogen mustards, sulphonate esters and cyclic nitrogen compounds), which prevents DNA and RNA synthesis, or by covalent binding to proteins (nitrosoureas), which blocks DNA repair processes.
When alkylating drugs bind to DNA nucleotides, such as to guanine, the alkylated nucleotide may either be repaired in which case the cell survives, or it may interfere with DNA replication by:
Because of the covalent nature of the product, these effects are not cell cycle-specific (Fig. 52.2). Alkylating agents are used to treat a wide variety of leukaemias, lymphomas and solid tumours.
Pharmacokinetics: The pharmacokinetic characteristics of the alkylating drugs depend on the nature of the reactive group(s) and the third non-reactive substituent on the N atom. Cyclophosphamide is an orally active prodrug that undergoes metabolic activation to produce two toxic metabolites, acrolein and phosphoramide mustard. Ifosfamide metabolism is similar to that of cyclophosphamide, and toxic metabolites of both cyclophosphamide and ifosfamide are excreted in the urine. Melphalan and chlorambucil, which have an aromatic substituent, undergo rapid metabolism. Most alkylating agents have half-lives of less than 6 h, but the duration of action on DNA is very long.



Cytotoxic antibiotics
Examples
anthracyclines: doxorubicin, epirubicin
other antibiotics: bleomycin, dactinomycin, mitomycin, mitoxantrone
Mechanisms of action and uses: The cytotoxic antibiotics have diverse chemical structures.


Cytotoxic antibiotics have several possible mechanisms of action.


In general, the mechanisms of action are not cell cycle-specific, although some members of the class show greatest activity at certain phases of the cycle, for example S phase (doxorubicin, mitoxantrone), G1 and early S phase (mitomycin), and G2 phase and mitosis (bleomycin).
Cytotoxic antibiotics have a wide spectrum of activity and are used for treatment of several leukaemias and lymphomas, as well as some solid tumours.
Pharmacokinetics: The cytotoxic antibiotics are poorly absorbed from the gut and are given intravenously. They are eliminated by metabolism and some have very long half-lives (mostly 12 h or longer).
Unwanted effects: Many of these drugs have radiomimetic properties. They should not be used at the same time as radiotherapy, since toxicity can be greatly increased.

Platinum compounds
Mechanism of action and uses
The platinum drugs enter cells and generate a reactive complex that crosslink guanine units in DNA. The result is similar to the effect of alkylating drugs by breaking the DNA chain. Cisplatin and carboplatin are used for ovarian and lung tumours. Cisplatin is also used for several other solid tumours. Oxaliplatin is used for advanced colorectal cancer.
Pharmacokinetics
These drugs are given by intravenous infusion and are mainly excreted by the kidney as platinum compounds. Cisplatin and oxaliplatin have long half-lives (24–60 h), largely owing to extensive protein binding.
Antimetabolites
Mechanism of action and uses: An astute clinical observation that the administration of folic acid to children with leukaemia exacerbated their condition led to the development of a folate antagonist, methotrexate. This represented an important landmark in cancer chemotherapy.
Folic acid in its reduced form (tetrahydrofolic acid, THF) is an important biochemical intermediate. It is essential for synthetic reactions that involve the addition of a single carbon atom during a biochemical reaction, such as the introduction of the methyl group into thymidylate and the synthesis of the purine ring system. During such reactions, THF is oxidised to dihydrofolic acid (DHF), which has to be reduced by dihydrofolate reductase back to THF before it can accept a further one-carbon group and be reused.
Methotrexate has a very high affinity for mammalian dihydrofolate reductase and inhibits its active site. This blocks purine and thymidylate synthesis and inhibits the synthesis of DNA, RNA and protein. It may show selectivity for cancer cells because these rely more on de novo synthesis of purines and pyrimidines, whereas normal tissues use salvage pathways that reutilise preformed purines and pyrimidines to a greater extent. Methotrexate is specific for S phase and slows G1 to S phase.
Methotrexate is given for acute lymphoblastic leukaemia, non-Hodgkin’s lymphomas and various solid tumours. It is also used an immunosuppressant at lower doses in non-malignant conditions such as inflammatory joint diseases and psoriasis. The mechanism of its immunosuppressant effect is different to its anti-cancer actions (Ch. 38).
Pharmacokinetics: Methotrexate is well absorbed from the gut but can also be given intravenously or intrathecally. It is eliminated by renal excretion, but a small amount may be retained for longer periods both strongly bound to dihydrofolate reductase and intracellularly as polyglutamate conjugates.
Toxicity is increased in the presence of reduced renal excretion, and methotrexate should be avoided if there is significant renal impairment. Folinic acid (leucovorin) is frequently administered shortly after high-dose methotrexate to reduce mucositis and myelosuppression. Non-steroidal anti-inflammatory drugs such as aspirin can reduce the renal excretion of methotrexate and increase its toxicity.
Base analogue antimetabolites
Examples
purine antagonists: fludarabine, gemcitabine, mercaptopurine, tioguanine
pyrimidine antagonists: capecitabine, cytarabine, fluorouracil, raltitrexed, tegafur
Mechanism of action and uses: A number of useful chemotherapeutic drugs have been produced by simple modifications to the structures of normal purine and pyrimidine bases (Fig. 52.4). These act in a number of ways to interfere with DNA synthesis, typically following intracellular phosphorylation and the incorporation of the triphosphate product into DNA or RNA. Detailed mechanisms are given for each drug in the Compendium at the end of the chapter. Base analogue antimetabolites are used for a wide variety of leukaemias, lymphomas and solid tumours.
Pharmacokinetics: Base analogues are mainly absorbed and metabolised by the pathways involved in absorption and metabolism of the corresponding unmodified base. Oral absorption is often erratic and most are given intravenously. The urine is a minor route of elimination (up to 1% of the parent drug) and most half-lives are in the range 1–8 h. Tegafur is a prodrug of fluorouracil and is given in combination with uracil, or with gimeracil and oteracil, which inhibit the breakdown of fluorouracil.


Mitotic inhibitors
Mechanism of action and uses: The vinca alkaloids are complex natural chemicals isolated from the periwinkle plant (Vinca rosea). Vinca alkaloids bind to tubulin and inhibit polymerisation and therefore assembly of microtubules, thus producing M-phase arrest of mitosis. They are therefore cycle-specific. Microtubules are essential for numerous cellular functions, including maintenance of cell shape, motility, transport between organelles and cell division.
The vinca alkaloids are used for various lymphomas and for acute leukaemia. They are also effective in some solid tumours.
Pharmacokinetics: Vinca alkaloids are usually given intravenously. Elimination is largely by metabolism with little renal excretion. They have very long half-lives.
Unwanted effects: The spectrum of unwanted effects differs between various drugs, despite their close structural similarities.

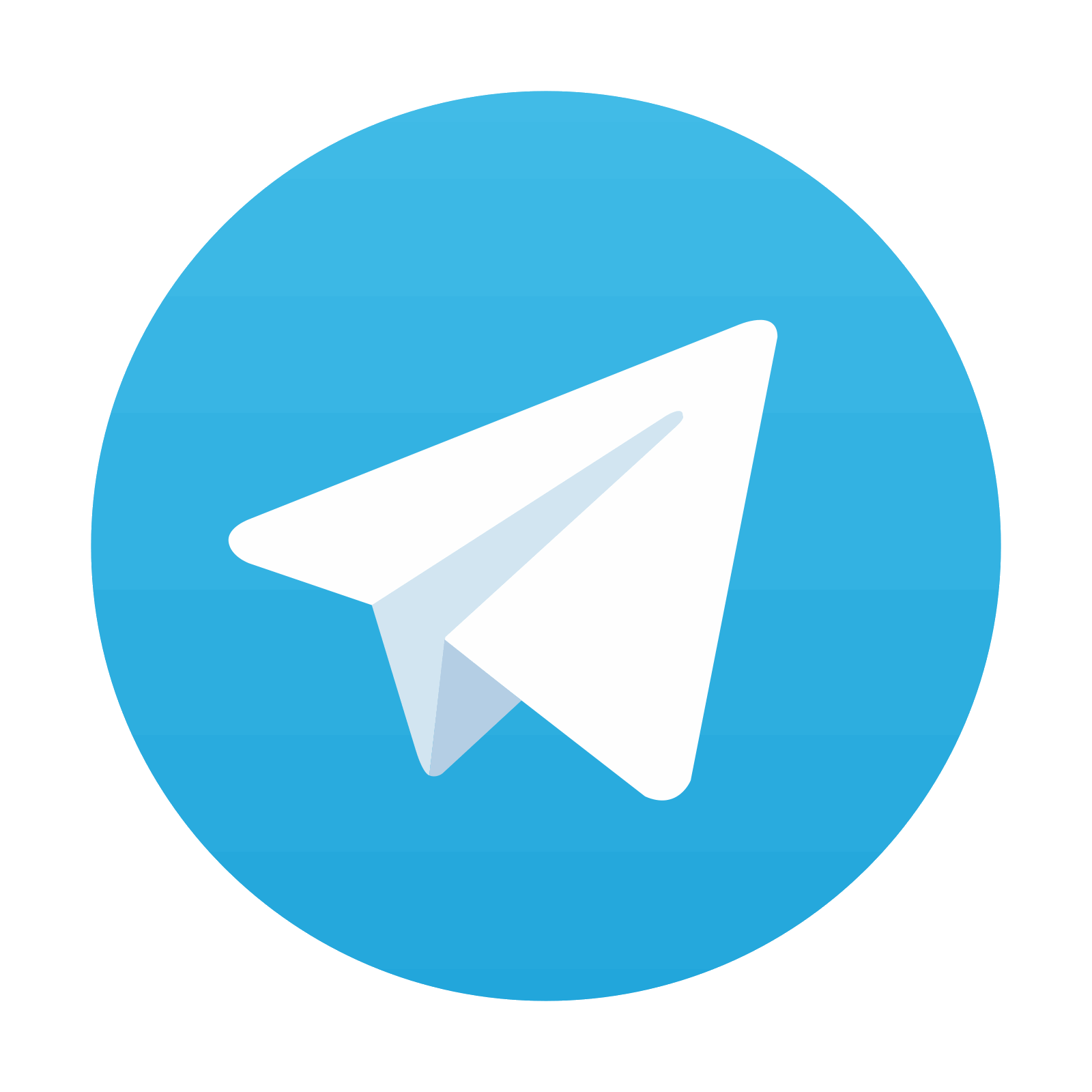
Stay updated, free articles. Join our Telegram channel
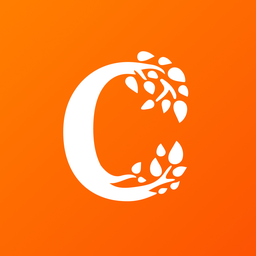
Full access? Get Clinical Tree
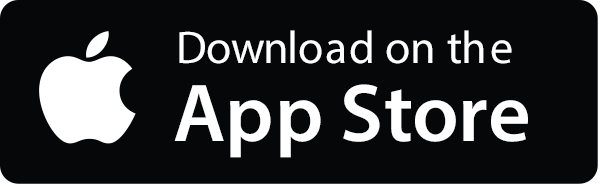
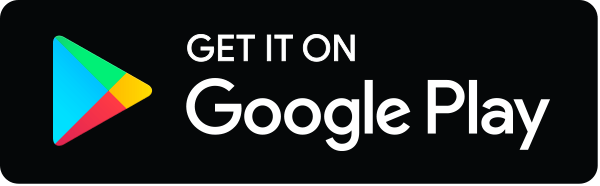
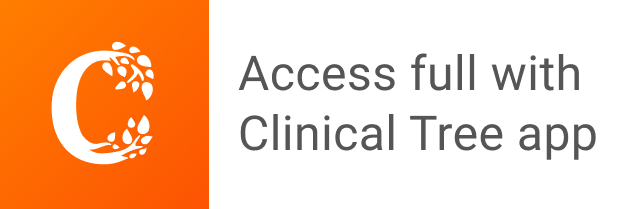