Fig. 1
Relationship between water solubility of TIHs and pulmonary target sites. Depending on the water solubility different primary target sites of TIHs in the human respiratory system can be determined. Highly water-soluble substances (e.g. ammonia, formaldehyde, sulfur dioxide) will affect mainly the upper respiratory system (A). Substances of intermediate solubility (e.g. ozone, chlorine) will affect tracheal and bronchial regions (B), whereas water insoluble substances (e.g. phosgene, oxides of nitrogens) will interfere with the lower respiratory system down to the alveolar spaces (C) (Bonner 2008, modified) (Shusterman 1999; Tuorinsky and Sciuto 2008)
Nevertheless, depending on the concentration of the TIH and time of exposure, chemicals can compromise the entire respiratory tract (Miller and Chang 2003; Tuorinsky and Sciuto 2008). As gases or vapors move by convection into the peripheral airways and then by diffusion to the alveolar-capillary membranes, TIHs reaching the alveolar space may have more profound health effects, because clearance due to gas exchange is much slower in this compartment (Urbanetti 1997). Pre-existing diseases of the airway system or damaged lung tissue due to former toxic inhalation injury may already compromise normal physiological lung function. In a mouse model of allergic asthma it was shown that additional inhalation of chemicals can enhance allergic airway inflammation in presensitized mice (Ban et al. 2006). Moreover, obstructive lung diseases (asthma, chronic bronchitis) can further constrain the elimination of TIHs leading to very severe health effects (Urbanetti 1997).
It is important to note that early clinical symptoms of airway irritation or damage do not always correlate with the clinical outcome or long-lasting health effects. Moreover, some TIHs may provoke serious health effects (e.g. pulmonary edema) after a latency period that may last several hours, during which signs and symptoms may not be evident, although cell damage has already occurred. Some chemicals have to undergo intracellular biotransformation processes before causing cell toxicity. It is remarkable that biotransformation is generally considered as a detoxification or defense process, but during this process, toxic metabolites may occur that can enhance lung injury (e.g. transformation of benzo(a)pyrene into reactive metabolites) (Cohen 1990; Smith and Brian 1991). If clinical symptoms arise instantaneously after exposure to certain TIHs, already existing target structures (e.g. cell-surface receptors) are affected and induce a cellular response. The biological effect depends on the activated sensor. In the case of stimulated nerve endings, fast cellular responses are most likely and are a typical example for ion channel activation after a toxic stimulus inducing acute pain.
TIHs with similar chemical properties (e.g. chemical reactivity) are likely to interact with similar cellular targets. The cellular response and the resulting tissue lesions are based upon the molecular function of the affected cellular targets. Thus, TIHs with similar reactivity will provoke comparable or identical clinical symptoms, although their chemical structure may differ. Moreover, if specific cellular targets and the cellular response of certain TIHs are known, the pathophysiology of related substances can be predicted to a certain degree. Numerous studies have been conducted to assess the mode of action of toxic reactive inhalants. Unfortunately, the mode of action of most toxicants is largely unknown and the clinical picture of intoxication is generally nonspecific, which complicates diagnosis and therapy. Therefore, detailed knowledge about the molecular toxicology of lung irritants is required to develop specific therapeutic strategies.
The biological response of the respiratory system after exposure to TIHs is a result of complex interactions of multiple factors, including the intensity of exposure (concentration and time), condition of exposed tissues and also protective and regenerative mechanisms (Urbanetti 1997). Toxic effects can be mediated by direct interaction of the TIHs and respiratory cells leading to cellular dysfunction or cell death. Moreover, chemical substances may activate afferent nerve endings immediately provoking clinical symptoms. Inflammatory mediators, chemokines and interleukins released from affected lung epithelial cells, stimulated macrophages or other cell types will trigger additional biological effects such as cellular infiltration, vasodilation and leakage, pulmonary edema, mucus production, repair and remodeling processes.
3 Clinical Symptoms of TIH Exposure Related to the Nasopharynx
Usually the nasal mucosa, nasopharynx and larynx are exposed to the highest concentrations of toxicants as these structures represent the first line of defense after inhalation. Typical first symptoms will occur due to mucosal irritation like a tickle of the throat and cough which are both biological alert signs with the aim of initiating an escape reaction from the dangerous environment. Coughing and sneezing are triggered by activation of peripheral sensory nerve endings in the nasal airway lining. These nerve endings can be considered as a first defense mechanism against chemical challenges (Bessac and Jordt 2008, 2010). The purpose of this initial reflex-response is the removal of irritants from the nasal epithelial surface. After activation of nasal trigeminal, glossopharyngeal or vagal chemosensory neurons (type C and small diameter myelinated Aδ fibres) by TIHs, sensory-autonomic pathways elicit parasympathetic reflexes, mediated via cholinergic efferent neurons, resulting in secretions from nasal, lacrimatory and salivary glands in combination with the dilatation of vessels in the nasal mucosa and sinuses (Bessac and Jordt 2008, 2010). Mucosal swelling and leakage are common results leading to narrowing or obstruction of the nasal passage (Baraniuk and Kim 2007). The resulting edema of the larynx and glottis can be life-threatening due to acute obstruction of the upper airways.
4 Clinical Symptoms of TIH Exposure Related to the Tracheobronchial System
When irritants reach the tracheobronchial section, sensory neuronal activity can trigger tracheal and bronchial constriction via contraction of bronchial smooth muscle cells, mucus secretion, and neurogenic inflammation (Fig. 2), events which are considered as typical reactions after exposure to lung irritants (Miller and Chang 2003). Rhonchus is the typical diagnostic finding resulting from mucous secretion and constriction of the bronchial musculature. TIHs can be removed from the respiratory tract by different mechanisms. The airways of the lower respiratory tract are lined with cells that are predominantly ciliated or mucus producing. TIHs are trapped within the mucus layer and are removed by ciliated cells via mucociliary transport. Macrophages, either integrated in the epithelium or macrophages in the alveoli, are capable of phagocytosis and thereby clearing inhaled particles. Macrophages migrate to upper parts of the respiratory tract, where they are trapped in the mucociliar transport and are cleared from the airways. Moreover, activated macrophages release cytokines, chemokines, growth factors, proteolytic enzymes and ROS. These mediators recruit and activate other cells that either participate in tissue regeneration or may contribute to tissue remodeling processes. After exposure to lung irritants, mucociliary clearance may increase when small or moderate concentrations of toxic chemicals are inhaled. However, high concentrations or very aggressive chemical substances may also interfere with the cilia of the respiratory epithelium or influence the epithelial integrity itself. As shown in an in vitro human bronchial co-culture cell culture model, sulfur mustard induced a widening of intercellular spaces and moreover, a loss in cell-matrix adhesion molecules was observed resulting in a decrease of transepithelial resistance (Pohl et al. 2009). Mucin production increased with resultant cessation of ciliar beat.


Fig. 2
Schematic section of a bronchus. Left part shows the physiological state, right part illustrates the (patho)physiological response after TIH exposure. Exposure with TIHs can lead to smooth muscle contraction (A) resulting in a reduction of airway diameter. Moreover, the epithelium lining the respiratory system and endothelium of pulmonary vessels can be affected, resulting in dysfunction, increased production of mucus (B) and fluid leakage into the aerial lumen (C), enhancing the reduction of airway diameter
5 Clinical Symptoms of TIH Exposure Related to the Alveolar System
Chemical substances with little water solubility or high concentrations of TIHs are able to reach deep lung compartments, e.g. the alveoli. The complex damaging processes of TIHs in the alveolar space are depicted in Fig. 3 and include liberation of inflammatory mediators, invasion of inflammatory cells, hypersecretion, and disturbance of cell-cell contacts leading to the destruction of the endothelial-epithelial barrier.


Fig. 3
Schematic illustration of the alveolar space and the cellular effects after TIH exposure. After exposure to TIHs, complex injury to lung tissue may occur. Damage to endothelial cells accompanied with epithelial damage, leads to alveolar edema and release of chemo-attractants. Inflammatory cells (e.g. monocytes, neutrophils) migrate from the blood vessels into the alveolar space, secreting interleukins thus inducing and maintaining inflammation. Proteinases are activated, enhancing cellular damage. Mucin production by secretory cells is enhanced. Growth factors are secreted, inducing repair and/or remodeling processes
Apparently, type I alveolar epithelial cells (covering 90–95 % of the alveolar surface) appear to be most vulnerable to many lung toxicants probably because of their large surface area. Dysfunctional alveolar type I cells have to be replaced by new type I cells originating from type II alveolar cells. Alternatively, fibroblasts are activated to repair tissue lesions resulting in the formation of connective tissue and scars. Chemicals that affect both alveolar type I and type II cells have to be considered as extremely harmful, as regenerative cells are compromised and tissue damage cannot be adequately repaired. As the blood-air barrier is extremely thin (~0.4–1 μm), toxic substances may penetrate into lung capillaries (especially after damage to alveolar type I cells) and affect the endothelium, which is comparably vulnerable as epithelial cells type I. This damage will result in progressive impairment of the blood-air barrier and permeability will increase. Tight and adherence junctions between cells are loosened, facilitating loss of epithelial resistance. As a result, blood plasma enters from lung capillaries into the lung interstitium. The resulting interstitial edema increases the diffusion distance through the blood-air barrier leading to disturbance of gas exchange. First, edema fluid is evacuated from the lung interstitium by the lymphatic system. Ongoing loss of epithelial and endothelial resistance will increase plasma leakage, overstraining the lymphatic system and resulting in alveolar edema. A pathological cycle is initiated: formation of edema foam, ascending from the alveolar space to the upper parts of the respiratory system, blocking bronchioles and impairing oxygenation. These events then cause increased pulmonary resistance which leads to further leakage into the alveoli. The whole process may result in a toxic lung edema that may be fatal.
Toxic lung edema due to increased alveolar permeability is hard to control, because tissue architecture is affected or even destroyed. Recovery of the integrity of the alveolar epithelial surface is an essential strategy after toxic inhalation injury. Especially, alveolar type I cells are vulnerable and may undergo cell death mediated either by direct TIH effects or indirectly by specific cytokines released from affected cells, residual structural cells or activated macrophages. Various acute inflammatory mediators are expressed in the lung during acute lung injury (e.g. IL-1α, IL-8, TNF-α, C3b, leukotrienes). In parallel, proteases are released from infiltrating neutrophils and mononuclear cells and degrade the extracellular matrix. Resulting debris is subsequently cleared by macrophages (attracted and differentiated from bone marrow and circulatory pools). Next, growth factors (e.g. TGF-ß, CTGF, PDGF) stimulate basal cells (type II alveolar cells) to differentiate into type I cells and other cell types reconstitute connective tissue, vascular tissue, and nerve endings. A careful balance of catalytic and anabolic processes is mandatory for a complete physiological repair. If one of the processes is gaining an upper hand, fibrosis due to enhanced production of collagen and connective tissue or emphysema due to enhanced protease activity may occur (Bonner 2008).
6 Members of the TRP Channel Family as Chemosensory Molecules in the Airways
Virtually all pathophysiological processes mentioned above are regulated or modulated by activation of chemosensory molecules in the plasma membrane of cells in the respiratory system. In particular chemosensory TRP channels play a pivotal role in the detection of TIHs, in the control of adaptive responses, and in the initiation of detrimental signaling cascades.
TRP channels represent one of the largest superfamilies of ion channels in the human genome (Clapham et al. 2001; Montell et al. 2002; Nilius and Owsianik 2011; Pedersen et al. 2005). Mammalian TRP channels can be subdivided into six families, i.e. TRPC, TRPM, TRPV, TRPP, TRPML, and TRPA channels as shown in Fig. 4. The common structural features of the members of the TRP superfamily are six transmembrane-spanning domains (TM1-6) and a putative pore region (P) located between transmembrane domains 5 and 6 (see Fig. 5). TRP channels are permeable to cations with striking differences in ion selectivity. Many TRP channels conduct monovalent cations like Na+ or K+ as well as bivalents like Ca2+, Mg2+ or bivalent cations of transition metals. Some TRP channels like TRPM4 and TRPM5 show high selectivity for monovalent cations, other channels like TRPV5 or TRPV6 are highly selective for Ca2+ (for review: Owsianik et al. 2006).



Fig. 4
Phylogenetic tree of TRP channels. The relationships between the TRP channels are illustrated by the branch lengths

Fig. 5
Common structural features of TRP channels. TRP channels have six transmembrane regions connected by intra- and extracellular loops. A loop between transmembrane domain 5 and 6 is essential for the formation of a pore in a functional TRP channel tetramer at the plasma membrane. PM plasma membrane, TM1-6 transmembrane-spanning domains 1–6, P pore region
The archetypal TRP channel, eponymous for the whole super-family, is the product of the trp gene of the fruit fly, Drosophila melanogaster. Cloning of the Drosophila TRP protein was preceded by the characterization of a mutant fruit fly with a visual defect. In this mutant strain, termed transient receptor potential (TRP) (Minke 1977; Montell et al. 1985), exposure to light only led to a short-lived depolarizing current due to an inactivating mutation of the TRP channel, which is an indispensable component of the phototransduction cascade in Drosophila (Hardie and Minke 1992; Montell et al. 1985; Montell and Rubin 1989). In some analogy to Drosophila TRP, many mammalian TRP channels exert sensory functions, although mostly outside of the visual system. Thus, TRP channels like TRPV1 (Caterina et al. 1999; Cesare et al. 1999; Tominaga et al. 1998), TRPV2 (Caterina et al. 1999), TRPV3 (Peier et al. 2002b; Smith et al. 2002; Xu et al. 2002), and TRPV4 (Guler et al. 2002; Watanabe et al. 2002) were reported to be activated by elevated temperature and are involved in thermo-sensing (for review: Tominaga 2007), whereas TRPM8 is activated by cold temperatures (McKemy et al. 2002; Peier et al. 2002a). Other TRP channels like TRPM5 are part of the signal transduction pathway engaged by taste receptors (Perez et al. 2002; Zhang et al. 2003). Of note, TRPM5 channel activity also depends on temperature (Talavera et al. 2005), a fact that explains why sweet or bitter food has a more intense taste in higher temperatures. Furthermore, TRP channels like TRPV4 react to mechanical stimuli in various cell types and organs (for review: Liedtke 2007; Plant and Strotmann 2007; Yin and Kuebler 2010) including the lung vasculature (Jian et al. 2008).
The most important function of TRP channels in the context of this review is their role as chemosensory molecules. In the respiratory system, especially TRPV1, TRPV4, TRPA1, and TRPM8 are involved in the detection of potentially harmful inhalants. However, these channels display a remarkable promiscuity with regard to their activators. For example, TRPA1 is activated by a large number of chemically unrelated substances (Table 1). Furthermore, TRP channels of the TRPC family are expressed in the lung and form part of redox-regulated signaling pathways. The activation of these TRP channels by TIHs results either from covalent modification of cystein residues of the ion channel or from phospholipase C (PLC)-regulated changes in lipid messengers (diacylglyercol, phosphatidyl-inositolphosphate) or intracellular calcium levels. In the first scenario, the TRP channel can be activated directly by reaction with an electrophilic TIH (Brone et al. 2008; Hinman et al. 2006; Macpherson et al. 2007a) or the reaction of the TIH with constituents of the plasma membrane leading to the generation of secondary TRP activators (Taylor-Clark et al. 2009; Trevisani et al. 2007). Regarding PLC-promoted signaling events leading to the activation of TRP channels, either stimulation of Gq/11-coupled protease-activated receptors (PARs) or purinergic receptors with subsequent activation of PLC-β or stimulation of redox-sensitive PLC isoforms is involved (see below).
Table 1
Examples of different TRPA1 activators with EC50 values (for human TRPA1) and putative activation mechanism.
Substance | EC50 | Putative mechanism | Literature |
---|---|---|---|
2-Pentenal | ? | Covalent cysteine modification | Bautista et al. (2006) |
Acetaldehyde | 77 μM | Covalent cysteine modification | Bang et al. (2007) |
Acrolein | 1-5 μM | Covalent cysteine modification | |
Allyl isothiocyanate (AITC) | 2–11 μM | Covalent cysteine modification | |
Chlorobenzylidene malononitrile (CS, tear gas) | 0.2 μM | Covalent cysteine modification | Brone et al. (2008) |
Crotonaldehyde | 16 μM–5.1 mM | Covalent cysteine modification | |
Formaldehyde | 200–357 μM | Covalent cysteine modification | |
Methylisocyanate | 25 μM | Covalent cysteine modification | Bessac et al. (2009) |
Ammonium chloride | 9.2 mM | pH shift | |
Nicotine | >10 μM | Non-covalent | Talavera et al. (2009) |
Isoflurane | 180 μM | ? | Matta et al. (2008) |
Hydrogen peroxide | 23–297 μM | Oxidation of cystein residues | |
Hypochlorite anion | Equivalent to 7–11 ppm of chlorine | Oxidation of cystein residues | Bessac et al. (2008) |
Of note, some pulmonary TRP channels are involved in O2 sensing by detecting hypoxic as well as hyperoxic states (for review: Takahashi et al. 2012). Hypoxia can lead to an activation of TRPA1- or TRPC6-promoted calcium responses (Fuchs et al. 2011; Takahashi et al. 2011; Weissmann et al. 2006). TRPA1 acting as an O2 sensor is expressed in vagal neurons in the lung and its activation by hypoxia occurs via reduced activity of proline hydroxylases (PHDs). In normoxia PHDs oxidize a proline residue in TRPA1 (Pro394) thus inhibiting the channel (Takahashi et al. 2011). Hyperoxic states lead to the direct oxidation of cystein residues of TRPA1 (Cys633 and Cys856), a modification, which overrides the inhibition of TRPA1 by proline hydroxylation (Takahashi et al. 2011). Whereas TRPA1 is directly modified by the ambient O2 concentration, TRPC6 is activated by hypoxic states via the enhanced generation of reactive oxygen species during hypoxia, which in turn lead to an accumulation of diacyl glycerol (DAG) (Weissmann et al. 2006). Thus, TIHs leading to obstruction of the airways via bronchoconstriction or increased mucus secretion can indirectly activate TRPA1 or TRC6. In contrast, oxidizing TIHs could potentially mimick hyperoxic conditions and by this means directly activate TRPA1.
To explain the role of TRP channels in pulmonary responses upon exposure to TIHs in more detail, this review will first focus on the involvement of TRP channels in irritative responses of the upper respiratory tract. Afterwards, we will discuss the special role of TRPA1 and TRPV1 in the cough reflex. Thereafter, the impact of TRP channels, especially TRPV4, in the regulation of mucociliary clearance will be addressed. Finally, the role of members of the canonical family of TRP channels, TRPCs, in redox-regulated pathways will be discussed.
7 TRP Channels and Irritative Responses in the Upper Respiratory Tract
As stated above, the mucosa of the eyes and the mucosa of the nasopharynx are first-line target of TIHs, especially those with high water solubility. Of note, all features of mucosal responses after exposure to TIHs, i.e. lacrimation, sternutation, nasal discharge, and irritative pain, are regulated by TRP channels.
Irritant-Induced Lacrimation
With regard to lacrimation responses several TRP channels are involved. TRPM8, TRPA1, and TRPV1 are known to be functionally expressed in nerve terminals in the cornea (Murata and Masuko 2006) and prototypical TRP agonists like menthol (TRPM8), allyl isothiocyanate (TRPA1), and capsaicin (TRPV1) lead to increased lacrimation (Parra et al. 2010). Of note, TRPM8, which is activated by cool temperatures, appears not to be involved in lacrimation caused by irritants, but to regulate basal tearing rate via a cooling effect of the evaporating tear film (Parra et al. 2010). Consequently, low concentrations of the TRPM8 agonist menthol led to increased tear flow in mice without inducing nocifensive effects, whereas high concentrations of menthol induced nocifensive behavior in a TRPM8-independent manner (Robbins et al. 2012). Apart from the aforementioned TRP channels expressed in corneal nerve endings, other thermo- and osmosensitive TRPV channels, i.e. TRPV2, TRPV3, and TRPV4 have also been described in corneal epithelial cells (Mergler et al. 2011). However, their (patho)physiological functions in the eye still await further clarification. Of note, a non-TRPM8 and non-TRPA1 component is involved in tear flow excited by drying of the cornea, which leads to increased osmolarity of the tear fluid (Hirata and Oshinsky 2012). Thus, especially the osmosensitive TRPV4 channel could be involved in the regulation of basal tear flow. Regarding the role TRP channels in irritant-induced lacrimation, TRPV1 and TRPA1 play the predominant role. Thus, well known lachrymators like constituents of tear gas (e.g. 2-chlorobenzalmalononitrile, CS), pepper spray (capsaicin) or onions (allicin) exert their irritative effects via activation of TRPA1 or TRPV1 (Salazar et al. 2008).
Role of TRPA1 and TRPV1 in Nasal Irritation by TIHs
Irritants acting on the nasal mucosa lead to a stimulation of trigeminal C fiber nerve endings with subsequent irritative responses. Many well-known trigger substances of these trigeminal reflexes, like capsaicin, menthol, or mustard oil directly target TRP channels. Capsaicin, for instance, has been extensively characterized as a tool to investigate airway reflexes mediated via C fibers (Coleridge and Coleridge 1977; Coleridge et al. 1965; Fuller et al. 1985). Application of capsaicin or other TRPV1 agonists lead to the release of substance P or CGRP by nerve endings in the nasal mucosa (Lundberg et al. 1984; Lundblad et al. 1983; Malis et al. 2001; Petersson et al. 1989) and thus, contributes to neurogenic inflammation in the nasopharynx. Functional studies have suggested that TRPV1, TRPA1, and TRPM8 are expressed in trigeminal neurons innervating the nasal cavity (Damann et al. 2006; Frasnelli et al. 2011; Karashima et al. 2007). Thus, these chemosensory channels have been postulated as critical sensor molecules in the nasopharynx involved in the detection of oxidizing or electrophilic TIHs (Bessac et al. 2008; Gerhold and Bautista 2009).
Interestingly, TRPV1 expression in the nasal mucosa has been also demonstrated in non-neuronal cells, e.g. in epithelial cells, vascular endothelium, and submucosal glands (Seki et al. 2006). Furthermore, stimulation of human cells from nasal or bronchial epithelium with capsaicin led to the production of interleukin-6 (IL-6) which could be blocked by co-treatment with TRPV1 antagonist capsazepine (Seki et al. 2007).
Cough Reflex: Role of TRPA1 and TRPV1
The cough reflex is an important danger signal upon exposure to harmful inhalants and protects the airways by removing ingested stimuli. However, chronic cough severely impacts the quality of life and represents an important, but often intractable reason for patients to consult a physician. Therefore, novel therapeutic options to treat chronic cough are urgently needed. In this context, it is important to note that cough can be induced via two distinct mechanisms, i.e., either by chemical irritation stimulating a reflex mediated via C fibers or by mechanical stimuli, which produce a cough reflex via Aδ fibers (for review: Brooks 2011). Whereas the role of TRP channels in mechanical induced cough is still not clearly defined, the role of these channels in chemically-induced cough is well established. Especially, TRPA1 and TRPV1 channels represent pivotal factors in regulating irritant-induced cough (for review: Bessac and Jordt 2008; Geppetti et al. 2010; Materazzi et al. 2009) and are promising therapeutic targets (for review: Banner et al. 2011; Preti et al. 2012).
Thus, TRPA1 agonists like allyl isothiocyanate (AITC) and TRPV1 agonists like capsaicin are potent inducers of cough reflex. Vice versa, TRPA1- and TRPV1- inhibitors have been shown to block cough responses provoked by exposure to the respective agonist in animal models (Brozmanova et al. 2012; Grace et al. 2012). In a comparative study using the guinea pig model, the TRPV1 activator capsaicin was more efficient to induce cough than the TRPA1 activator AITC (Brozmanova et al. 2012). Regarding potential endogenous activators of the TRP-promoted cough reflex, it has been shown that prostaglandin E2 (PGE2) and bradykinin (Bk) can induce a tussive response in animal models and are able to activate TRPA1 and TRPV1 in human, mouse and guinea pig airway cells (Grace et al. 2012). These findings are commensurate with previous results showing that Bk can activate TRPA1 via a PLC- and cAMP-dependent protein kinase (PKA)-dependent mechanism (Bandell et al. 2004; Wang et al. 2008). Interestingly, the contribution of these channel in Bk- or PGE2-promoted cough was additive: thus, the sole use of TRPV1- and TRPA1-blockers led to partial inhibition of the tussive response to both stimuli, whereas combination of TRPV1 and TRPA1 inhibitors completely abrogated cough responses (Grace et al. 2012). While the exact activation mechanism of TRPA1 and TRPV1 by PGE2 and Bk is not fully elucidated, these findings indicate that a number of exogenous or endogenous processes leading to the release of inflammatory mediators including Bk and PGE2 could potentially lead to an activation of these cough-associated TRP channels.
The importance of human TRPs in the pathogenesis of cough has been recently investigated in a genetic analysis of single nucleotide polymorphisms (SNPs) of TRPV1 and their relationship with cough symptoms in 844 asthmatic and 2,046 non-asthmatic patients (Smit et al. 2012). Of note, seven SNPs of TRPV1 showed a statistically significant association with cough in non-asthmatics (six SNPs with nocturnal cough and one with usual cough). Furthermore, four SNPs of TRPV1 were associated with an increased risk of cough in persons (asthmatics and non-asthmatics) with exposure to irritants (Jugg et al. 2011). For other TRP channels like TRPV4 and TRPA1 no significant relationship of SNPs with cough could be detected in this study.
These findings suggest that TRPV1 plays a crucial role in mediating chemically-induced cough-responses. However, with regard to reactive, electrophilic inhalants like acrolein, croton aldehyde, methyl isocyanate, or AITC, TRPA1 may be more important as primary target. As stated above, electrophilic and oxidizing compounds are able to activate TRPA1 as well as TRPV1 via covalent modification of cystein residues (Hinman et al. 2006; Macpherson et al. 2007a; Salazar et al. 2008). However, the electrophilic α, β-unsaturated aldehyde acrolein activated C fibers in wild-type and TRPV1-knockout mice, a response that could not be modified by additional treatment with TRPV1 blocker iodoresiniferatoxin indicating that at least in this murine model TRPV1 is not the primary target of a prototypical electrophilic inhalant (Symanowicz et al. 2004). The importance of TRPA1 in response to electrophilic inhalants is further supported by findings in guinea pigs, which presented pronounced coughing upon challenge with AITC, cinnamaldehyde, acrolein, or croton aldehyde, which could be blocked by inhibition of TRPA1 using the selective antagonist HC-030031 but not by inhibition of TRPV1 using the selective blocker capsazepine (Andre et al. 2009).
TRPV4 and Mucociliary Clearance
As mentioned before, a critical function of the bronchial system involved in adaptive responses to exposure to TIHs is related to the mucociliary clearance system. The driving force for increased ciliary activity in the bronchial system is an increase of the intracellular calcium concentration in ciliary cells (Salathe and Bookman 1995). Therefore, chemosensory, calcium-permeable channels of the TRP family are plausible candidates to modulate ciliary beat frequency after chemical irritation. In fact, TRPV4 expression has been described in many ciliary cells, e.g. in the ovarian duct (Andrade et al. 2005), in cholangiocytes (Gradilone et al. 2007), and also in bronchial epithelial cells (Lorenzo et al. 2008; Seminario-Vidal et al. 2011).
In ciliated cells of the oviduct treatment with high viscosity fluid increased ciliar activity, an effect that could be blocked by loading cells with TRPV4 antibodies and was mimicked by TRPV4 agonists (Andrade et al. 2005) indicating that TRPV4 is a major component of mechanically induced ciliary activity in these cells. In contrast, TRPV4 appeared not to be critically involved in ciliary activity upon viscous challenge in bronchial epithelium, since highly viscous fluid did not provoke different effects in tracheal epithelial cells from wild-type or TRPV4-knock-out mice (Lorenzo et al. 2008). However, stimulation of tracheal cells with ATP, a well-known activator of ciliary beat frequency in the bronchial system, led to a receptor-operated calcium signal strongly dependent on TRPV4 (Lorenzo et al. 2008). Intriguingly, activation of TRPV4 has been described to stimulate the release of ATP by airway epithelial cells induced by cell swelling in a pathway involving pannexin 1, RhoA and myosin light chain phosphorylation (Seminario-Vidal et al. 2011).
These findings suggest that TRPV4 and ATP may form part of a self-amplifying system with TRPV4 responsible for ATP-promoted calcium signaling and subsequently triggering ATP release after activation (Fig. 6). Moreover, ATP release by airway cells has been demonstrated after treatment with the lung toxicant bleomycin and has been proposed to form a universal danger signal (Riteau et al. 2010).


Fig. 6
Involvement of TRPV4 in ATP-signaling in bronchial epithelium. Stimulation of metabotropic purinoceptors (e.g. P2Y2) leads to the activation of TRPV4 which in turn can trigger the release of ATP. By this means, the interplay of ATP-dependent G protein coupled receptors and TRPV4 may sustain a self-amplifying signaling cascade with high extracellular levels of ATP representing a danger signal in lung epithelial cells
8 Pulmonary TRPC Channels
In recent years evidence for an involvement of TRPC channels in lung function and toxicity became evident. Although TRPC channels are not the primary sensor molecules in different lung tissues, they are essential members of signal transduction cascades mediating toxicity in the targeted cells. TRPC channel activity is induced after ligand binding to a receptor (e.g. a Gq/11 protein-coupled receptor) and complex intracellular signaling cascades (Dietrich et al. 2005).
The seven TRPC family members can be grouped into subfamilies on the basis of their amino acid sequence similarity. While TRPC1 and TRPC2 are almost unique, TRPC4 and TRPC5 share ~65 % identity. TRPC3, 6 and 7 form a structural and functional subfamily with 70–80 % identity at the amino acid level and their ability to be activated by DAG (Dietrich et al. 2005; Hofmann et al. 1999; Okada et al. 1999), a product of phosphatidylinositol 4,5-bisphosphate cleavage by activated phospholipases C. In all eukaryotic cells, activation of phospholipase C (PLC)-coupled membrane receptors by hormones or neurotransmitters leads to an increase in the intracellular Ca2+ concentration [Ca2+]i. Both activation of PLCβ and ε-isozymes by G protein coupled receptors and of PLCγ isoforms by receptor tyrosine kinases results in the hydrolysis of phosphatidyinositol 4,5-bisphosphate and the generation of inositol 1,4,5-trisphosphate (IP3) and DAG. While it seems clear that TRPC3/6/7 channels are directly activated by DAG and are responsible for receptor-operated calcium entry (ROCE) (Hofmann et al. 1999; Okada et al. 1999), the regulation of other members and their involvement in store operated calcium entry (SOCE) is discussed controversially. SOCE occurs when inositol 1,4,5-trisphosphate (IP3) or some other signal discharges Ca2+ from intracellular stores in the endoplasmic reticulum (ER). The subsequent fall in the ER Ca2+ concentration then signals to the plasma membrane and activates store-operated channels. A major breakthrough in understanding SOCE was the identification of the calcium sensor STIM (Liou et al. 2005; Roos et al. 2005; Zhang et al. 2005) and the Orai channels (Cahalan 2009; Feske et al. 2006; Vig et al. 2006) in 2005 and 2006, respectively. STIM 1 which is predominantly located in the ER, is a single transmembrane domain protein containing two N-terminal Ca2+-binding EF hands in the ER lumen and is therefore ideally situated to detect calcium levels in the ER. Upon Ca2+ release reduced calcium levels induce STIM redistribution to punctae and opening of store-operated channels in the plasma membrane. The so called Orai proteins with only four predicted transmembrane domains and intracellular C- and N-termini, were identified as the pore forming unit of store-operated channels by the analysis of T cells from patients with severe combined immunodeficiency (SCID) reviewed in Cahalan (2009). Although Orai 1 channels can multimerize with themselves to form pore forming units, it was also suggested that Orai molecules might interact with TRPC channels heterologously expressed in HEK293 cells to form store-operated channels (Liao et al. 2009). Moreover, evidence supporting the interaction of the calcium sensor STIM1 with TRPC proteins was presented for the same heterologous expression system (Yuan et al. 2009a, b).
TRPCs are also regulated by phosphorylation. TRPC3 channels can be inactivated by direct phosphorylation of amino acids threonine 11 (Thr 11) and serine 263 (Ser 263) by protein kinase G (PKG) (Kwan et al. 2004) and by protein kinase C (PKC)-mediated phosphorylation of Ser 712 (Trebak et al. 2005). The receptor tyrosine kinase Src phosphorylates TRPC3 at Tyrosine 226 (Tyr 226) and inhibits TRPC3 activity (Kawasaki et al. 2006), while Fyn another member of the Src family increases TRPC6 activity (Hisatsune et al. 2004). It has been shown that TRPC6 is negatively regulated after phosphorylation of Thr 69 by PKG (Takahashi et al. 2008b). Most interestingly, this phosphorylation is essential for anti-hypertrophic effects of phosphodiesterase 5 inhibitors (Kinoshita et al. 2010; Koitabashi et al. 2010; Nishida et al. 2010), which are also important therapeutics for pulmonary hypertension (Reichenberger et al. 2007).
TRPC channels can form functional homo- and heterotetramers within two defined subgroups (TRPC1/4/5 and TRPC3/6/7) excluding TRPC2 which is a non-functional pseudogene in humans (Goel et al. 2002; Hofmann et al. 2002). Novel combinations of TRPC1- with TRPC4 or 5 together with TRPC3, 6 or 7 in tetrameric complexes with three different TRPC family members have also been identified in HEK293 cells, as well as in embryonic brain, but not in adult rat tissues (Strubing et al. 2003). Evidence for the importance of the highly conserved pore region in the TRPC tetramer is derived from site-directed mutagenesis which results not only in the complete loss of channel activity upon heterologous expression, but also in a dominant-negative effect of a mutated channel monomer on functional homo- or heteromeric channel tetramers (Hofmann et al. 2002).
The TRPC family includes seven members (TRPC1-7), but only six are functional in humans, because TRPC2 is a pseudogene in the human genome. TRPC7 expression in lung is sparse and is predominantly identified in alveolar macrophages (Finney-Hayward et al. 2010). Expression of the other TRPC channels in pulmonary tissues and recent evidence concerning their potential function is summarized in the next paragraph.
9 Role of TRPC1, TRPC3, TRPC4, and TRPC5 in the Lung
Although TRPC1 was the first mammalian TRP channel to be cloned, its physiological functions are still elusive. It is not even clear, if TRPC1 is an ion channel or merely an adaptor protein regulating ion channel activity of other TRPC members (Storch et al. 2012). Homomeric TRPC1 complexes do not translocate to the plasma membrane (Hofmann et al. 2002), but TRPC1 as a member of heteromeric TRPC1/5 or TRPC1/4 complexes has a high impact on TRPC4/5 currents (Strubing et al. 2001) and decreases calcium permeability in such channel complexes (Storch et al. 2012). It has been reported that TRPC1 is important for the proliferation of bronchial smooth muscle cells, which may induce thickening of the airways facilitating airway hyperreactivity (summarized in Li et al. 2003). Along these lines, TRPC1 is up-regulated in proliferating airway smooth muscle cells while TRPC1 down-regulation inhibits cell proliferation (Sweeney et al. 2002a, b). However, it is not clear, if proliferation is influenced exclusively by TRPC1 channels or by TRPC1/4 channel complexes, because heteromeric TRPC1/4 channel complexes seem to be more important for the lung extra-alveolar endothelial barrier than for the capillary endothelial barrier (summarized in Cioffi et al. 2009). TRPC3 expression is up-regulated by tumour necrosis factor (TNF)-α in human airway smooth muscle cells (White et al. 2006). TNF-α is a pro-inflammatory cytokine which has been implicated in asthma and COPD because it increases airway hyperresponsiveness (AHR) by increasing airway smooth muscle Ca2+. Therefore, TNF-α blockage might represent a promising pharmacological target for the treatment of asthma and COPD (summarized in Amrani 2007). The function of TRPC4 in lung vascular endothelial cells was analysed using TRPC4−/− mice and wild-type controls. A defect in thrombin-induced Ca2+ influx is associated with reduced actin stress fiber formation and reduced endothelial cell retraction response. In isolated perfused lungs thrombin receptor activation increased the microvessel filtration coefficient (Kfc) by 2.8-fold in wild-type lungs but only 1.4 in TRPC4−/− lungs. This data provides evidence for a role of TRPC4 together with other ion channels in lung endothelial permeability (Tiruppathi et al. 2002). Recently TRPC5 was detected in the apical membrane of neuroepithelial bodies in the intrapulmonary airway epithelium by TRPC5 specific antibodies. However, it remains elusive if and how TRPC5 is involved in signal transduction cascades of these cells (Lembrechts et al. 2012).
10 TRPC6: Involvement in Redox-Sensitive Pathways in the Lung
Many studies were already published on the role of TRPC6 channels in the airways. Indeed, TRPC6 function in the lung might be particularly important, because it is the most prominently expressed TRPC channel in lungs (Hofmann et al. 2000). Therefore, we set out to analyze allergic responses in a TRP6-deficient mouse model expecting a reduced response in comparison to wild-type (WT) mice. However, we found an increased methacholine-induced AHR in TRPC6-deficient mice compared to WT mice. This surprising finding is most probably due to compensatory up-regulation of TRPC3 in airway smooth muscle cells (Sel et al. 2008). By experimental inflammation induced by intraperitoneal ovalbumin (OVA) sensitization followed by OVA aerosol challenges we were able to detect a decreased level of T-helper type 2 (Th2) cytokines (IL-5 and IL-13) as well as reduced IgE levels in the blood of TRPC6−/− mice compared to WT mice. Mucus production in goblets cells from challenged mice however was not altered in TRPC6-deficient mice (Sel et al. 2008). This data points to an important role of TRPC6 in the immune responsive rather than in airway smooth muscle tissues. Because neutrophil numbers are increased in the sputum of COPD and asthma patients, TRPC6 function in these cells might be also important for the progress of the diseases. Migration of TRPC6−/− neutrophils in response to macrophage inflammatory protein-2 (MIP2 also known as CXCL2) was reduced compared to WT neutrophils (Damann et al. 2009). In the same report an involvement of TRPC6 in cytoskeletal rearrangements during neutrophil migration was demonstrated suggesting an important role of TRPC6 in the migration of lung neutrophils. TRPC6 was also identified in other immune cells of the lung like alveolar macrophages (Finney-Hayward et al. 2010). Most interestingly, TRPC6 mRNA expression was significantly increased in macrophages obtained from COPD patients compared to healthy controls, while TRPC3 and TRPC7 levels remained unchanged (Finney-Hayward et al. 2010).
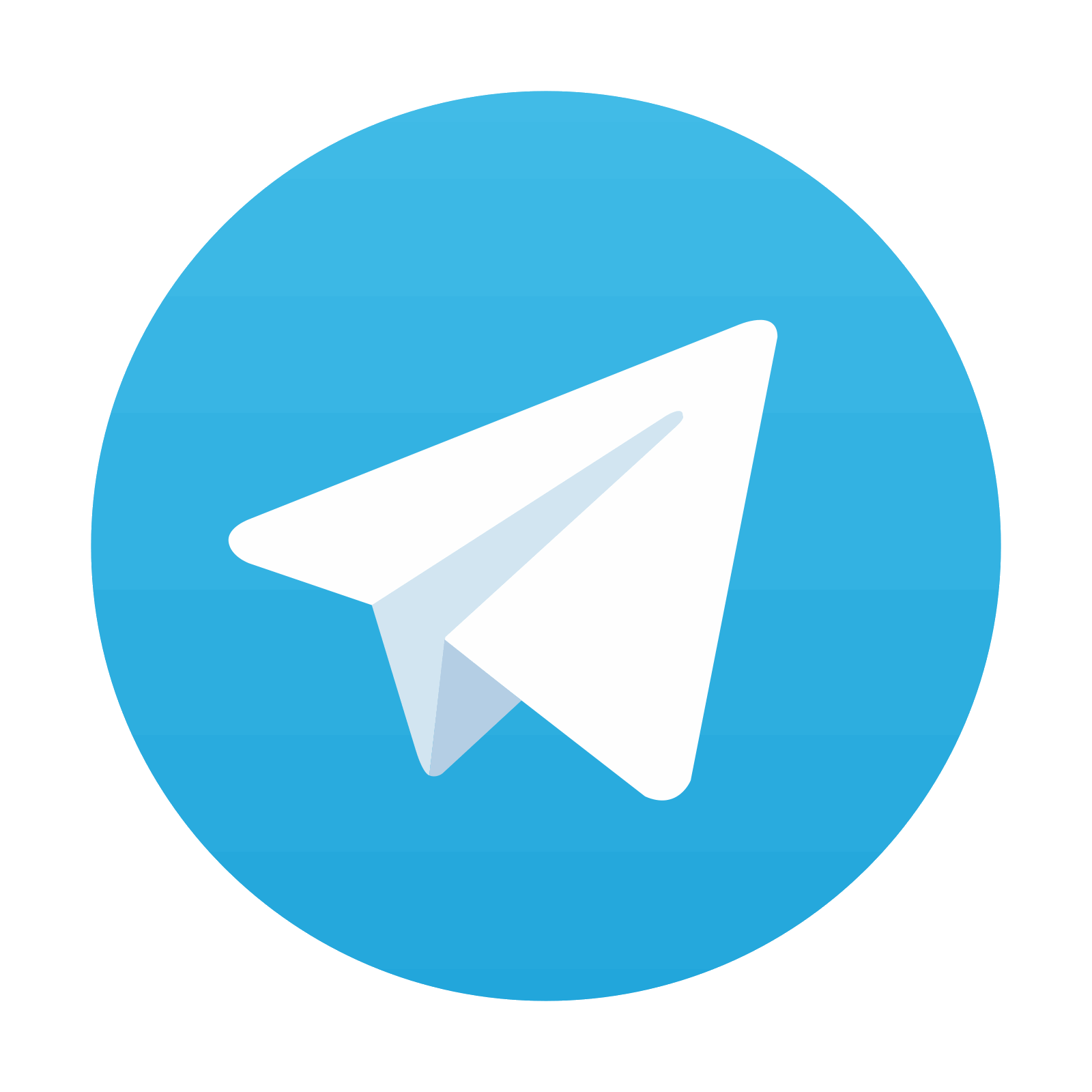
Stay updated, free articles. Join our Telegram channel
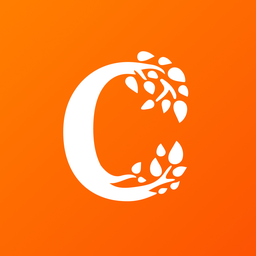
Full access? Get Clinical Tree
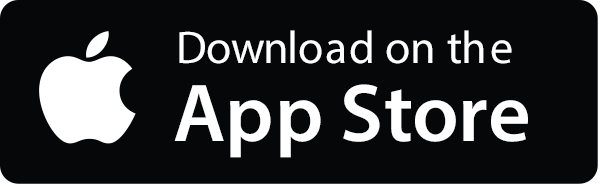
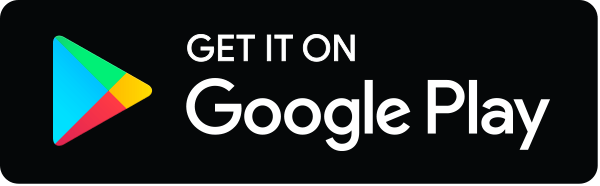