An ideal nerve conduit should have characteristics of biocompatibility, biodegradability, semi-permeability, ability for axon regeneration, and easy handling. There are three types of the nerve conduits. The first is synthetic scaffolds including silicone, polyglycolic acid, poly l-lactic acid, poly-3-hydroxybutyrate and polytetrafluoroethylene. The second is natural polymers such as chitosan, silk fibroin, collagen, chitin and gelatin. The third is natural conduits such as vein and artery grafts which are better for maintaining cell viability, supporting proliferation and permitting intercellular communication and growth factor elution [15, 27, 43, 44, 63]. For the present, several nerve tubes have been approved by US Food and Drug Administration for human uses, based on type I collagen (Neuragen®, Neuroflex™, NeuroMatrix™, NeuraWrap™, NeuroMend™), porcine small intestinal submucosa (Surgis® Nerve Cuff), poly(glycolic acid) (Neurotube®) and poly(d,l-lactide-co-e-caprolactone) (Neurolac®) [10, 51], but they are not recommended for gaps larger than 3 cm. In addition to their supportive role, the nerve conduits also help prevent unwanted cell dissipation from the injured site.
By realizing the importance of basal lamina and ECM framework for axonal guidance, the nerve conduits with different internal structure composed of multiple fibers have become more popular than hollow tubes. Recent advances in the design of internal structure of the nerve conduit focus on topographical features of guiding axonal regeneration and cell migration, based on the concepts of large interior channels to separate individual fiber fascicles, scaffolds with many longitudinally orientated pores, and parallel aligned polymer fibers produced by melt extrusion or electrospinning. Recently, the biodegradable polyester poly(E-caprolactone) (PCL) was electrospun into microfibers, then embedded in collagen gels and incorporated parallel in PCL tubes. This composite conduit could guide the direction of SCs migration and axonal growth of embryonic chicken dorsal root ganglia [35]. It is suggested that packing density and distribution of intraluminal structures can influence nerve regeneration, for example, higher densities (approx. 15–30 % of the cross-sectional area) of poly(l-lactide) (PLLA) microfilaments inhibit nerve regeneration, while lower densities (approx. 3.75–7.5 % of the cross-sectional area) enhance nerve regeneration [13].
The morphology and behavior of the cell are different according to the different fiber thickness. The poly(lactic-co-glycolic acid) (PLGA) fibers with 700 nm diameter increase olfactory-ensheathing cells (OECs) attachment with rounded cells in random orientation, whereas culture on 250 nm fibers enhance a unidirectional alignment with a characteristic bipolar shape. After seeding on silk fibroin nanofibers, migration, morphology, adhesion, spread, gene and protein expression of OECs could be influenced and modulated by the content and structure of these nanofibers. OECs with polygonal morphology could be observed on short nanofibers, on longer and parallel alignment nanofibers, OECs presented characteristic bipolar shape with more extensive migration properties [17]. 1800 nm silk fibroin scaffolds made OECs randomly disperse, however 300 nm scaffolds with the native fibrils of the ECM induced OECs to display a superior alignment and a better migration [54].
The natural silk from silkworm consists primarily of two major proteins: sericin and fibroin. Sericin is responsible for the cell adherence and has an important role for regarding nerve regeneration, but sericin is well known for inducing immunoreactivity. Up to the present, there are no study for comparing silk from silkworm (fibroin) and spiders (spidroins) or artificially prepared silk proteins. The different silk may has its own advantages and disadvantages for the spatial structures, cell seeding and release of bioactive molecules, which may have an important influence on the three-dimensional cell assembly, cell migration and axon regeneration.
Previously, the nerve conduits are mainly used for bridging the nerve defects of 2 cm or less, but it is a challenge to bridge larger nerve defects. Recently, Radtke et al. [50] adopted acellularized veins filled with spider silk to bridge a 6-cm peripheral nerve defect in adult sheep and showed that regenerated axons within the graft were found 8 months after the lesion and myelinated by endogenous invading SCs, followed by an improved electrophysiological and locomotor function that was comparable to that of autologous nerve transplantation.
2.2 Delivery Methods
Delivery method involves how to give the contents like the donor cell and the muscle into the lumen of the nerve conduit (Fig. 2). Generally, the donor cells can be directly injected within the conduit lumen or can be seeded onto the conduit matrix. If the autograft or allograft nerve is going to be used for peripheral nerve repair, the donor cells suspended in culture medium can be microinjected into ends of the grafts. However this delivery method can be traumatic to the delicate intra-neural architecture and can result in unpredictable cell distribution. The vein and artery grafts being rich in ECM proteins like collagen and laminin can provide a useful substrate for cell adhesion. Vessel grafts can remain empty or can be pre-filled with tissue such as muscle to support axonal guidance. Usually commercially available natural conduits are composed of ECM components such as collagen and fibrin. The degradation profile of the conduits should be carefully considered when used for cell transplantation. Natural conduits can degrade in a predictable, non-toxic fashion, but their degradation rate may not be sufficiently slow to allow for adequate regeneration time. Some synthetic polymers can acidify the microenvironment during degradation, so can result in a detrimental impact on cellular activity [16].
Recently, it has also been evidenced that systemic administration of the donor cells can be effective for peripheral nerve repair. With further refinement, this delivery method may become possible to sustain regenerative support through regular systemic dosing. It has also been confirmed that the donor cells can be effective when administered at the site of neuromuscular junction or at denervated muscle [12]. A comparative study of the delivery way has recently been performed by intramuscular and intravenous injection of BMSCs after rat PNI with small gap neurorrhaphy, the results shown that the targeted muscular injection resulted in much superior outcomes compared to the intravenous injection, evidenced by increases in the sciatic function index, nerve conduction velocity, myelin sheath thickness and restoration rate of gastrocnemius muscle wet weight [61].
2.3 Oxygen Supply
For long nerve conduit a major problem is the oxygen supply within the tube, which can affect the survival and function of the filled cells and be a limiting factor for the repair of long nerve defect. To increase the oxygen supply, perfluorotributylamine (PFTBA) is used as a synthetic oxygen carrier and combined into a collagen-chitosan conduit filled with OECs for repairing 15-mm-long rat sciatic nerve defect. The survived OECs are more in number in the PFTBA-enriched tubes than in the tubes with OECs alone, suggesting the beneficial effect of PFTBA on the OECs survival in vivo [67].
2.4 Clinical Trials
The synthetic nerve conduits have been used for the repair of human PNI in China [18, 23] and USA [29]. A chitosan/polyglycolic acid (PGA) nerve conduit is adopted for repairing a 35-mm-long median nerve defect at elbow of a human patient. During the 3-year follow-up period, functional recovery of the injured median nerve is assessed by pinch gauge test, hydraulic hand dynamometry, static two-point discrimination and touch test and electrophysiological examinations. This implantation improves the recovery of the motor and sensory function at M4 and S3+ levels [18]. In another case, 30-mm-long median nerve defect in the right distal forearm of a 55 year-old male patient is repaired with same synthetic nerve conduit, and results in the recovery of the palm abduction of the thumb and the thumb-index digital opposition. In addition, compound muscle action potentials is recorded on the right abductor pollicis and perspiration function of the injured thumb, index and middle fingers is partially recovered, indicated by the ninhydrin test which is a classical method for assessing sympathetic nerve function. These repair cases show that the synthetic nerve conduits are beneficial to the repair of larger defect of human peripheral nerve trunk in clinic.
3 ECM
ECM is an acellular component composed of proteoglycans (collagen and elastin) and fibrous proteins (fibronectin and laminin). It provides an environment for the survival, development and differentiation of the cells and the tissues. In PNS, ECM has profound influences on SCs behavior such as adhesion, differentiation, survival, growth, and migration. During the peripheral nerve regeneration, SCs migration from both proximal and distal nerve stumps into the site of the lesion will be limited without the formation of ECM cable.
Among ECM proteins, fibronectins and laminins are the most widely studied in peripheral nerve regeneration. Fibronectin is mainly synthesized and secreted by SCs and forms a fibrillar network in association with type IV collagens and laminins. Although it is expressed at relatively low levels in the SCs basement membrane of adult peripheral nerves, fibronectin is rapidly upregulated following nerve injury, either deposited from plasma or synthesized by fibroblasts and endothelial cells. During the development of the nervous system, fibronectin is mainly involved in the migration and differentiation of the neural crest cells and responsible for SCs adhesion, proliferation, and neurite outgrowth. Fibronectin knockout mice die early in gestation, and nervous system abnormalities are apparent, including incomplete closure of the neural tube. Laminin, a complex trimeric glycoprotein composed of one a, one B, and one y chain and ranged in size from 400 to 900 kDa, is a main protein for the maturation of the PNS and appears to be crucial for the SCs to successfully myelinate axons [21]. During the repair of PNI, this glycoprotein has positive effects on SCs adhesion, proliferation, migration, and the ability to improve nerve regeneration. It has been confirmed that the lack of laminin is manifested as reduced myelination, discontinuous basal lamina, and atypical SCs ensheathment.
In vitro cell culture laminin and fibronectin are usually added to the culture disk for coating. Although both have a ability for SCs proliferation and neurites sprout of dorsal root ganglia (DRG) neurons, the best outcome result from the synergistic action of fibronectin and laminin [20]. In vivo, laminin and fibronectin not only benefit to the behavior of glial and neuronal cells, but also promote stem cells differentiate into SC-phenotype. After adding ECM to the nerve conduits, ECM modified-scaffold can promote survival and migration of endogenous cells and transplant exogenous cells in the PNI repair.
Also ECM plays a role for selectively attaching and releasing growth factors such as brain-derived neurotrophic factor (BDNF), ciliary neurotrophic factor (CNTF), fibroblast growth factor (FGF), glial cell derived neurotrophic factor (GDNF), nerve growth factor (NGF), neurotrophin-3 (NT-3). For example, NT-3 and BDNF have shown good affinity to fibrinogen, whereas CNTF is easy to combine with laminin. This selective binding property of ECM can be benefit to the drug design and release.
4 Donor Cells
The ideal donor cell for peripheral nerve repair should be easily accessible, rapidly expandable in culture, capable of in vivo survival and integration into host tissue [16]. In addition the donor cell should be amenable to stable transfection and expression of exogenous genes.
4.1 Stem Cells
It has been widely demonstrated in vitro and in vivo that stem cells can replace lost neurons, increase the number of glial cells, rescue axotomized neurons, and manipulate the microenvironment of the neurons during regeneration. During regeneration of peripheral nerve, increasing number and activity of SCs is especially emphasized. Exogenous stem cells can differentiate into SC-like phenotype, form Büngner Bands, guide axon regeneration, and re-myelinate nerve fibers. After stem cells being transplanted, growth factor secretion and ECM production can be enhanced by direct releasing and paracrine signaling. Then, secreted factors stimulate endogenous SCs to upregulate secretory activity. ECM proteins such as collagen I, collagen IV, fibronectin and laminin have regenerative effects, but ECM components of chondroitin sulphate proteoglycan (CSPGs) is a potent inhibitor of axonal regeneration. However CSPGs can be cleaved from the basement membrane by activated matrix metalloproteinases [21], thus still providing a permissive ECM environment for axonal regeneration.
Both transplanted stem cells and endogenous SCs can produce NGF, BDNF, GDNF, CNTF and NT-3. Some angiogenic factors like vascular endothelial growth factor (VEGF), basic fibroblast growth factor (bFGF), hepatocyte growth factor (HGF) and angiopoietin-1 may also be released by the stem cells. In addition, the stem cells express leukemia inhibitory factor and insulin-like growth factor, which are potential to improve neuronal survival, promote corticospinal tract growth, increase astrogliosis and potentiate the inflammatory response . The immunomodulatory effects of the stem cells are believed to be due to the secretion of granulocyte and macrophage colony stimulating factor (G-CSF, M-CSF), interleukin -6, 7, 8, and 11 (IL-6, IL-7, IL-8, IL-11) and tumor necrotic factor-α (TNF-α). If the host immune response is properly suppressed, the detrimental impact of inflammation and fibrosis following injury can be reduced. The levels of ECM protein and growth factor production may vary not only with stem cell type but also with the differentiation status of stem cells [16].
The stem cells can be used in their undifferentiated state or SC-like cells differentiated in vitro by exposure to β-mercaptoethanol, all-trans retinoic acid, fetal bovine serum, forskolin, recombinant human bFGF, recombinant human platelet derived growth factor-AA, and recombinant human heregulin β-1. The benefits of differentiation include superior in vivo viability and an enhancement of neurotrophic factor secretion and myelinating ability. However, differentiation in vitro can incurs an unnecessary delay for clinical applicability, reduces the secretion of neurotrophic factors , maintains in vivo neuronal differentiation difficult. The undifferentiated cells may undergo in vivo differentiation in response to local stimuli, but have a risk of differentiation along unwanted non-neuronal lines [16].
4.1.1 Embryonic Stem Cells (ESCs)
ESCs isolated from blastocysts can form derivatives of all three embryonic germ layers (Fig. 3). These cells are homogenous, and can provide an unlimited source of cells with superior differentiation potential and long-term proliferation capacity. Their disadvantages include immunogenicity and tumourigenicity. Additionally, ethical controversy exists potentially due to the fact that cells are harvested during the blastocyst stage of development, which consequently result in the destruction of embryos [16].


Fig. 3
Different stem cell sources. ESCs are obtained from the inner cell mass of the blastocyst, therefore require destruction of the embryo. NSCs are harvested from the subventricular layer of the lateral ventricle and the subgranular layer of the hippocampus. BMSCs are harvested from the marrow cavity of long bones. ADSCs are derived from subcutaneous fat, which is abundantly available following liposuction. SKPs are harvested from the dermis and represent a related populations of cells harvested from hair follicles. FSCs can be obtained from amniotic membrane, amniotic fluid, umbilical cord blood, umbilical cord tissue and Wharton’s jelly. DPSCs can be harvested from deciduous teeth. Reproduced from Fairbairn et al. [16]
It has been shown that ESCs can differentiate into neurons and glial cells of the central and peripheral nervous system. The capacity for in vivo myelination also has been confirmed [6, 66]. Previous studies about application of ESCs to peripheral nerve repair are limited, perhaps reflecting the lack of facile clinical translatability [11], but few studies reported that after ESCs transplanted directly into muscle of small animal with sciatic and tibial nerve transection can preserve short-term muscle mass and myocyte cross-sectional area [37]. Realizing this limitation, investigators have derived mesenchymal precursors from ESCs. It has been reported that transplanting spheres of mesenchymal stem cells (MSCs) derived from ESCs can improve the peripheral nerve regeneration [39].
4.1.2 Nerve Stem Cells (NSCs)
NSCs are first isolated from adult murine brain in the early 1990s, followed by similar discoveries in humans and non-human primates [16]. The brain subventricular zone and subgranular zone represent the primary sites of NSCs differentiation in adult mammalians (Fig. 3). Populations of NSCs and neurogenesis have been found in adjacent areas of the adult human striatum [14]. Many studies have reported positive results following NSCs implantation into injured peripheral nerve (reviewed by Fairbairn et al. [16]). NSCs transplantation is not only beneficial to the acute PNI, but also beneficial to the chronically denervated nerve for its recovery of structure and function [28]. Unfortunately, transplantation of NSCs into rat models of crush and transection, neuroblastoma formation has been encountered [32]. At present, immortalized murine C17.2 NCSs are commercially available and are commonly used for in vivo animal studies, but there are not uniform positive outcomes experienced with C17.2 NSCs in the repair of PNI.
4.2 Mesenchymal Stem Cells (MSCs)
MSCs are multipotent stromal cells originating from the bone marrow and different non-marrow tissue such as adipose tissue, skin, hair follicle and dental pulp (Fig. 3). Originally the potency of these cells is considered to be limited to tissues of mesodermal origin. Now it is generally accepted that they can differentiate into non-mesodermal lineage. MSCs can differentiate in vitro into osteoblasts, chondrocytes, adipocytes, cardiomyocytes, hepatocytes and neural lineages, including neurones, astrocytes, oligodendrocytes, microglia and SCs. Here the main sub-types of MSCs and their application to peripheral nerve regeneration will be briefly discussed as following.
4.2.1 Bone Marrow Derived Stem Cells (BMSCs)
BMSCs are easily accessible from the marrow cavity of long bones without potential ethical concerns (Fig. 3). Therefore these cells are more clinically applicable than ESCs, NSCs and SCs. Under appropriate conditions BMSCs can differentiate into neurons, astrocytes and SC-like cells of non-mesodermal lineages. It has been shown that adding BMSCs to the nerve conduits and acellular grafts results in superior results when compared with empty or cell depleted channels. Although few studies are failed to show that BMSCs can match outcomes achieved with cultured SCs, the majority of reports demonstrate that performance is at least equivalent [4], even a dearth of studies have shown superiority over gold standard autograft, which may be dose dependent [52]. BMSCs generally have inferior proliferation capacity and differentiation potential with low stem cell fraction. Recently, BMSCs with Platelet-rich plasma filled into Neurolac is used to repair 1 cm-defect of the sheep radial nerve (sensory) and the tibial nerve (motor). After 6 months increased myelinated nervous fibers and conduction velocity can be observed from both repaired radial and tibial nerves [9]. As BMSCs harvest is invasive and painful, less invasive MSCs sources such as adipose tissue, skin, hair follicle and fetal tissue are adopted.
4.2.2 Adipose Derived Stem Cells (ADSCs)
ADSCs result from the adipose tissue, and is abundant and easily harvested from abundant subcutaneous fat tissue via small biopsies or conventional liposuction procedures (Fig. 3). Especially, ADSCs can enhance neovascularization in ischemic conditions by secreting endothelial growth factor (EGF). In comparison to BMSCs, ADSCs have superior proliferation and differentiation potential [58]. Unlike BMSCs, donor age and anatomical site of origin do not seem to significantly influence therapeutic effect of ADSCs [56]. Based on the expression of myelin protein zero, peripheral myelin protein and myelin basic protein by ADSCs, capability of ADSCs myelinating regeneration axons is kept. Although some studies report that ADSC-filled conduits have no difference in outcome compared to cell deplete products, several studies have shown that ADSCs are at least as effective as autologous SCs, even have equivalent and superior outcomes in comparison to autograft. By comparing the performance of ADSCs and BMSCs, no significant differences have been found [45]. Low harvest morbidity, wide availability and superior stem cell characteristics have made this stem cell to be preferred one for pre-clinical studies.
4.2.3 Skin Derived Precursors (SKPs)
SKPs from the dermis (Fig. 3) are easily expandable in culture, and can give rise to different cell types such as melanocytes, craniofacial cartilage, bone and connective tissue, smooth muscle of vasculature, endocrine cells, and neurons and glial cells of the autonomic and peripheral nervous system, which are similarity to embryonic neural crest cells. When cultured with neuregulin-1β, known to promote proliferation and differentiation of SCs from embryonic neural crest precursors and to mediate SCs proliferation during Wallerian degeneration, SKPs express markers consistent with functioning SCs. Both undifferentiated and differentiated SKPs can maintain differentiation and viability, myelinate axons, and exhibit superior outcomes following de-myelination and crush injury, acute and chronic transection injury [16]. SCs differentiated from skin-derived SC precursors (SKPSCs) are highly proliferative glial cells, can enhance functional recovery after acute and chronic PNI [34] by modulation of the immune response and enhancement of macrophage recruitment to injury sites for more efficient debris clearance through the expression of interferon-γ (IFN-γ), interleukin (IL)-1β, and IL-6 [57].
4.2.4 Fetal Derived Stem Cells (FSCs) [16]
Fetal tissue represents a promising alternative source of stem cells. FSCs can be harvested from fetal tissues such as amniotic membrane, amniotic fluid, umbilical cord cells, umbilical cord blood and Wharton’s jelly (Fig. 3). FSCs are readily expandable in culture and possess the ability to differentiate into neural phenotype. It has been confirmed that amniotic fluid derived stem cells (AFDSCs) possess characteristics of both MSCs and NSCs and can differentiate into neural tissue. Following transplantation into rat sciatic nerve gap models, AFDSCs have been shown to promote peripheral nerve regeneration, but its survival following transplantation is limited. Umbilical cord-derived stem cells have also been shown to improve outcomes following crush and transection injuries in rodent models. Wharton’s jelly derived stem cells can differentiate into functional SC-like cells, produce NGF, BDNF, NT-3 and stimulate neurite growth in vitro.
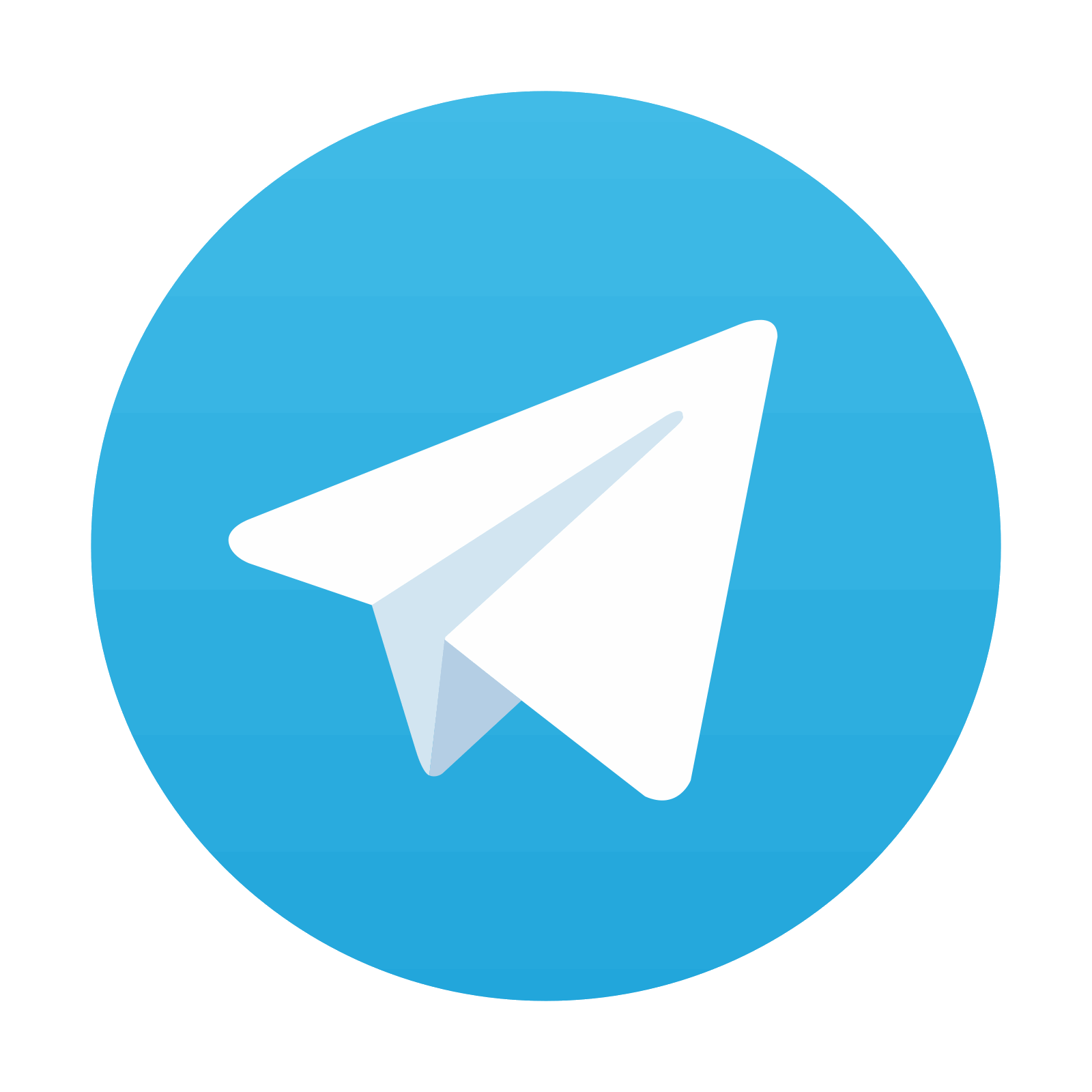
Stay updated, free articles. Join our Telegram channel
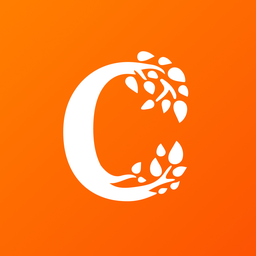
Full access? Get Clinical Tree
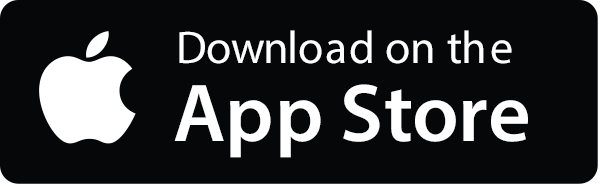
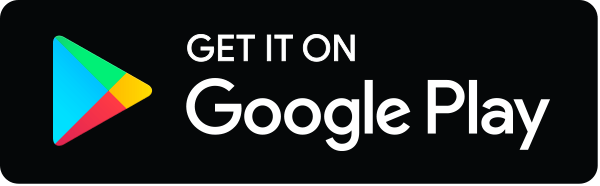