and Jürgen Roth2
(1)
Medical University of Vienna, Vienna, Austria
(2)
University of Zurich, Zurich, Switzerland
Central Nervous System: Neuron, Glial Cells
Providing the base for the most sophisticated functions and tasks of the body, including memory and creativity, the tissue of the nervous system has attracted the greatest attention from the earliest days of microscopy to the present. The complex functional processes in the central and peripheral parts of the nervous system are continuously topics in the life sciences and medical research. The identification and characterization of neural stem cells within certain areas of the adult brain has revolutionized our knowledge and understanding of adult neurogenesis, and has increased hopes for possible treatment and repair of damage resulting from injuries or degenerative diseases.
At first glance, electron micrographs of nerve tissue originating from the central nervous system, as shown in panels A and B, convey a confusing impression. It is difficult and perhaps impossible to identify every detailed structure visible, belonging to either of the two principal types of cells, nerve cells (neurons) or glial cells. At the left-hand side of panel A, the large body of a nerve cell can be seen, as well as one of its processes, a dendrite, projecting toward the upper right corner of the picture. The neighboring brain parenchyma consists of numerous, countless processes of both nerve and glial cells. The pictures in both panels make evident that the intercellular spaces are extremely narrow and intercellular structures are virtually lacking.
Neurons are the structural and functional units of the nerve tissue and show different functional domains. These include the cell body (soma), one process extending from the cell body and transmitting impulses away from the cell body, called a neurite or axon, one or more processes transmitting impulses from the periphery toward the cell body, called dendrites, and the synaptic junctions, where another neuron or an effector cell is contacted and the impulse transmitted (cf. Figs. 178 and 179). The nerve cell body (soma, panel A) contains the nucleus and the surrounding perinuclear cytoplasm, the perikaryon, where all components of the biosynthetic apparatus, ribosomes, endoplasmic reticulum, Golgi apparatus, and all organelles that maintain the cell, are present. A similar range of organelles is found in the dendrites. Although the axon hillock, the site where the axon (neurite) extends from the perikaryon, due to the plane of the section, is not visible in panel A, multiple axons can be seen in both panels. The micrographs show characteristic ultra-structures of brain parenchyma. Numerous profiles of axons are visible. They are grouped and sheathed by fine processes of glial cells, and contain mitochondria and abundant neurofilaments and microtubules that function as tracks for axoplasmic transport. In the right-hand segment of panel B, several axons are sheathed by myelin (asterisks; cf. Fig. 182), which in the central nervous system is produced by specialized neuroglial cells, the oligodendrocytes. Protoplasmic and fibrous astrocytes represent another class of glial cells. With their processes, they are intimately apposed to neurons, cover axons, build up superficial limiting layers at the boundaries to the pia mater, and form perivascular feet (cf. Fig. 178).
References
Bannai H, Inoue T, Nakayama T, Hattori M, Mikoshiba K (2003) Kinesin dependent, rapid, bi-directional transport in dentrites of hippocampal neurons. J Cell Sci 117:163
Gonzáles C, Couve A (2014) The axonal endoplasmic reticulum and protein trafficking: cellular bootlegging south of the soma. Semin Cell Developm Biol 27:23
Hickey WF, Vass K, Lassmann H (1992) Bone marrow-derived elements in the central nervous system: an immunohistochemical and ultrastructural survey of rat chimeras. J Neuropathol Exp Neurol 51:246
Horne J (2004) New neurons? Nat Cell Biol 6:287
Matteoli M, Coco S, Schenk U, Verderio C (2004) Vesicle turnover in developing neurons: how to build a presynaptic terminal. Trends Cell Biol 14:133
Priller L (2003) Grenzgänger: adult bone marrow cells populate the brain. Histochem Cell Biol 120:85
Sanai N, Tramontin AD, Quninones-Hinojosa A, Barbaro NM, Gupta N, Kunwar S, Lawton MT, McDermott MW, Parsa AT, Verdugo JM-G, Berger MS, Avlarez-Buylla A (2004) Unique astrocyte ribbon in adult human brain contains neural stem cells but lacks chain migration. Nature 427:740
Song H, Stevens CF, Gage FH (2002) Astroglia induce neurogenesis from adult stem cells. Nature 417:39
Wisco D, Anderson ED, Chang MC, Norden C, Boiko T, Fölsch H, Winckler B (2003) Uncovering multiple axonal targeting pathways in hippocampal neurons. J Cell Biol 162:1317
Zimmermann DR, Dours-Zimmermann MT (2008) Extracellular matrix of the central nervous system: from neglect to challenge. Histochem Cell Biol 130:635


Fig. 177
Magnification: ×5,500 (A), ×26,500 (B)
Blood–Brain Barrier, Synapses
For more than 100 years, it has been known that a specialized barrier protects the brain from harmful substances circulating in the blood. Different mechanisms contribute to the blood–brain barrier, which influence both paracellular and transcellular traffic. The endothelium is continuous, and extended tight junctions between the adjacent endothelial cells seal the intercellular spaces. Specific carrier mechanisms are involved even in the transport of small molecules, and the number of receptors is small. Furthermore, substances leaked into the brain parenchyma are transported back into the blood by multidrug resistance transporters present in the endothelial cells. The two neighboring vessels (asterisks) shown in panel A are characteristic of the brain microvasculature. They are lined with a continuous layer of endothelial cells. Transport vesicles are sparse, reflecting the restricted transepithelial transport. Perivascular cells, known to be derived from the bone marrow, accompany the vessels. Within the basal lamina (arrowheads), a pericyte can be seen localized close to the endothelium. Several cell bodies of astrocytes are apparent near the vessels, and numerous profiles of glial cell processes can be seen closely apposed to the basal lamina, forming a perivascular layer.
Panels B and C show chemical synapses, specialized adhesive sites for communication between two neurons, where impulses are conducted by neurotransmitters (cf. Fig. 179). A synapse consists of a presynaptic component that often is a terminal knob of an axon where synaptic vesicles (arrows) containing the transmitters are accumulated, and a postsynaptic component that often is a spine-shaped process of a dendrite. The specialized pre- and postsynaptic membranes are separated only by a 20–30 nm space, the synaptic cleft. Triggered by Ca2+-influx via voltage-gated channels, the transmitter is released into the synaptic cleft by fusion of the synaptic vesicle membrane with the presynaptic membrane (cf. Fig. 179). The transmitter diffuses across the synaptic cleft and is bound by the specific receptors concentrated in the postsynaptic membrane. As a consequence, ions are allowed to enter via ligand-gated channels, leading to changes of the polarization and causing either excitation or inhibition at the postsynaptic membrane. At the presynaptic neuron, transmitter release is rapidly followed by, either “kiss and run” or clathrin-mediated endocytosis, and transport to endosomal compartments. Under the electron microscope, layers of dense materials are visible, which are associated with the synaptic membranes, corresponding to their particular composition and organization. According to the favorite localizations, asymmetric and symmetric types of synapses have been discriminated. Asymmetric synapses (panel B), which correspond mainly to excitatory synapses with glutamate used as transmitter, show particularly prominent postsynaptic densities (arrowhead in B). Asymmetric synapses are further characterized by predominance of round synaptic vesicles (arrows in B). In contrast, symmetric synapses (panel C), which often correspond to inhibitory synapses, show prominent densities associated with both the pre- and postsynaptic membranes. In symmetric synapses, flattened synaptic vesicles predominate (arrows in C).
References
Dresbach T, Nawrotzki R, Kremer T, Schumacher S, Quinones D, Kluska M, Kuhse J, Kirsch J (2008) Molecular architecture of glycinergic synapses. Histochem Cell Biol 130:617
Hickey WF, Vass K, Lassmann H (1992) Bone marrow-derived elements in the central nervous system: an immunohistochemical and ultrastructural survey of rat chimeras. J Neuropath Exp Neurol 51:246
Holburg A, Lippoldt A (2002) Tight junctions of the blood-brain barrier: development, composition, and regulation. Vascul Pharmacol 38:323
Jarousse N, Kelly RB (2001) Endocytic mechanisms in synapses. Curr Opin Cell Biol 13:461
Matteoli M, Coco S, Schenk U, Verderio C (2004) Vesicle turnover in developing neurons: how to build a presynaptic terminal. Trends Cell Biol 14:133
Micheva KD, Buchanan J, Holz RW, Smith SJ (2003) Retrograde regulation of synaptic vesicle endocytosis and recycling. Nat Neurosci 6:925
Nitta T, Hata M, Gotoh S, Seo Y, Sasaki H, Hashimoto N, Furuse M, Tsukita S (2003) Size-selective loosening of the blood-brain barrier in claudin-5-deficient mice. J Cell Biol 161:653
Sytnyk V, Leshchynska I, Dityatev A, Schachner M (2004) Trans Golgi network delivery of synaptic proteins in synaptogenesis. J Cell Sci 117:381
Yamagata M, Sanes JR, Weiner JA (2003) Synaptic adhesion molecules. Curr Opin Cell Biol 15:621


Fig. 178
Magnification: ×6,600 (A); ×53,000 (B); ×82,000 (C)
Structure of the Synaptic Terminal
The transfer of information between neurons, or between neurons and other excitable cells, takes place at specialized junctions called synapses. In the mammalian central nervous system, most synapses function by converting electrical signals into chemical substances (neurotransmitters). Once released from the presynaptic terminal neurotransmitters bind postsynaptic receptors. Communication is unidirectional, resulting in the formation of very different structures at each side of the synapse (termed pre- and postsynaptic terminals, respectively). Synaptic terminals are separated by a ~ 25 nm gap known as the synaptic cleft (SC). Panel A shows a tomographic slice (2.7 nm in thickness) of a vitrified central nervous system synapse from the synaptosomal fraction.
Presynaptic terminals are specialized compartments that contain numerous neurotransmitter-filled synaptic vesicles (SV), as well as other organelles such as endoplasmic reticulum and mitochondria (MIT). Electrical signals (action potentials) are generated at the neuronal cell body and travel along the axon until they reach the presynaptic terminal. Action potential arrival causes a Ca2+ influx through voltage-gated Ca2+ channels that in turn stimulates synaptic vesicle fusion with the presynaptic membrane at the so-called active zone, a region of the membrane that directly faces the postsynaptic side and hosts the fusion machinery. After releasing the neurotransmitters, synaptic vesicles are endocytosed and prepared for the next round of release. Panel C3 shows a vesicle captured in the moment when its membrane is continuous with the plasma membrane.
As can be seen in panel B, synaptic vesicles (yellow) are extensively interconnected via filamentous bridges (red), while those located in the vicinity of the active zone (gray) are most often tethered to it by similar strands (blue). Details of these structures and their corresponding 3D renderings can be seen in panels C1 (connector) and C2 (tether).
The postsynaptic terminal is normally found on dendrites and it harbors a dense array of postsynaptic receptors and signaling and scaffolding proteins, the so called postsynaptic density (PSD). The effects of the neurotransmitter binding to postsynaptic ionic channels and receptors can be broadly separated in two categories. First, the ionic flux at the postsynapse is altered, resulting in excitatory or inhibitory potentials (depending on the synapse type) that change the excitability of the postsynaptic cell. Second, various signal transduction pathways can be initiated by the activation of second messengers leading to diverse effects, from modulation of synaptic strength to gene transcription. MT: Microtubule.


References
Biederer T, Stagi M (2008) Signaling by synaptogenic molecules. Curr Opin Neurobiol 18:261
Dalva MB, McClelland AC, Kayser MS (2007) Cell adhesion molecules: signalling functions at the synapse. Nat Rev Neurosci 8:206
Fernández-Busnadiego R, Asano S, Oprisoreanu A-M, Sakata E, Doengi M, Kochovski Z, Zürner M, Stein V, Schoch S, Baumeister W, Lučić V (2013) Cryo-electron tomography reveals a critical role of RIM1α in synaptic vesicle tethering. J Cell Biol 201:725
Haucke V, Neher E, Sigrist SJ (2011) Protein scaffolds in the coupling of synaptic exocytosis and endocytosis. Nat Rev Neurosci 12:127
Kennedy MJ, Ehlers MD (2006) Organelles and trafficking machinery for postsynaptic plasticity. Ann Rev Neurosci 29:325
Levitan EB, Kaczmarek LK (2002) The neuron. Oxford University Press
Neher E, Sakaba T (2008) Multiple roles of calcium ions in the regulation of neurotransmitter release. Neuron 59:861
Opazo P, Sainlos M, Choquet D (2012) Regulation of AMPA receptor surface diffusion by PSD-95 slots. Curr Opin Neurobiol 22:453
Saheki Y, De Camilli P (2012) Synaptic vesicle endocytosis. Cold Spring Harb Perspect Biol 4:a005645
Sheng M, Hoogenraad CC (2007) The postsynaptic architecture of excitatory synapses: a more quantitative view. Ann Rev Biochem 76:823
Südhof, TC (2013) Neurotransmitter release: the last millisecond in the life of a synaptic vesicle. Neuron 80:675

Fig. 179
Magnification: ×142,000 (A), ×260,000 (C1, C2, C3)
Unmyelinated Nerve Fiber
In the peripheral nervous system, nerve cell processes – both axons (neurites) and dendritic axons of sensory neurons – are surrounded by specialized glial cells, the cells of Schwann. The axon, its sheath of Schwann cells, and the surrounding basal lamina form the impulse conducting structures, the nerve fibers, classified in myelinated and unmyelinated types with respect to the presence or absence of a myelin sheath (cf. Fig. 182). Along myelinated nerve fibers, conduction of impulses is fast, because the myelin sheath forms an insulating layer and the impulse “jumps” from one intercellular region free of myelin to the next myelin-free region (cf. Fig. 183). In nerve fibers lacking a myelin sheath, the nerve impulses move continuously and less rapidly. Unmyelinated axons include most of the postganglionic axons from autonomic ganglia.
The unmyelinated nerve fiber shown in the electron micrograph belongs to the autonomic nervous system in the wall of the small intestine. Seven axons can be seen, either more superficially or more deeply invaginated in the cytoplasm of a cell of Schwann. The Schwann cell nucleus is visible in the right segment of the nerve fiber. In unmyelinated nerve fibers, one Schwann cell as a rule houses more than one axon. The Schwann cell cytoplasm forms thin envelopes around the axons, for each of which its own groove is provided. Schwann cells also produce the components of the basal lamina that covers the entire nerve fiber. Localized close to the axolemma (plasma membrane of the axon), the plasma membrane of the Schwann cell surrounds the axons, leaving a small but regular space. Most axons are completely engulfed by the Schwann cell. In these cases, the “lips of the grooves” are closed and a mesaxon (open arrows) is formed. In contrast, the axon at the left-hand side of the nerve fiber containing microtubules (arrows), a mitochondrion (asterisk), and accumulations of small synaptic vesicles is only partially enveloped by the cell of Schwann. Partly, its plasma membrane is devoid of a Schwann cell sheath and covered only by the basal lamina (arrowheads). Along unmyelinated nerve fibers, such areas with open lips and the axolemma exposed to the neighborhood are particularly prominent close to regions of special synapses where transmitters are delivered to diffuse to the surfaces of target cells, such as smooth muscle or secretory cells. Such a “synapse á distance en passant” is also shown in panel B of Fig. 175, where the axon is completely free of a Schwann cell sheath and the axolemma covered only by the basal lamina.
In the axoplasm (the cytoplasm of the axons), in addition to microtubules called neurotubules (arrows), abundant filaments including 10 nm thick neurofilaments, are visible. Both microtubules and neurofilaments provide tracks for axonal transport. Fast axonal traffic is bidirectional, requires an intact microtubule cytoskeleton, and involves motor proteins, such as kinesin and dynein. Kinesin is implicated in the anterograde transport, by which newly synthesized molecules and membrane-bound organelles, such as mitochondria and multiple vesicles, including synaptic vesicles, are carried from the perikaryon to the axon periphery. Dynein is involved in retrograde traffic from terminal regions of the axon to the perikaryon, which, in particular, is the route of substances and molecules internalized at the axon endings and is misused by viruses and toxins.
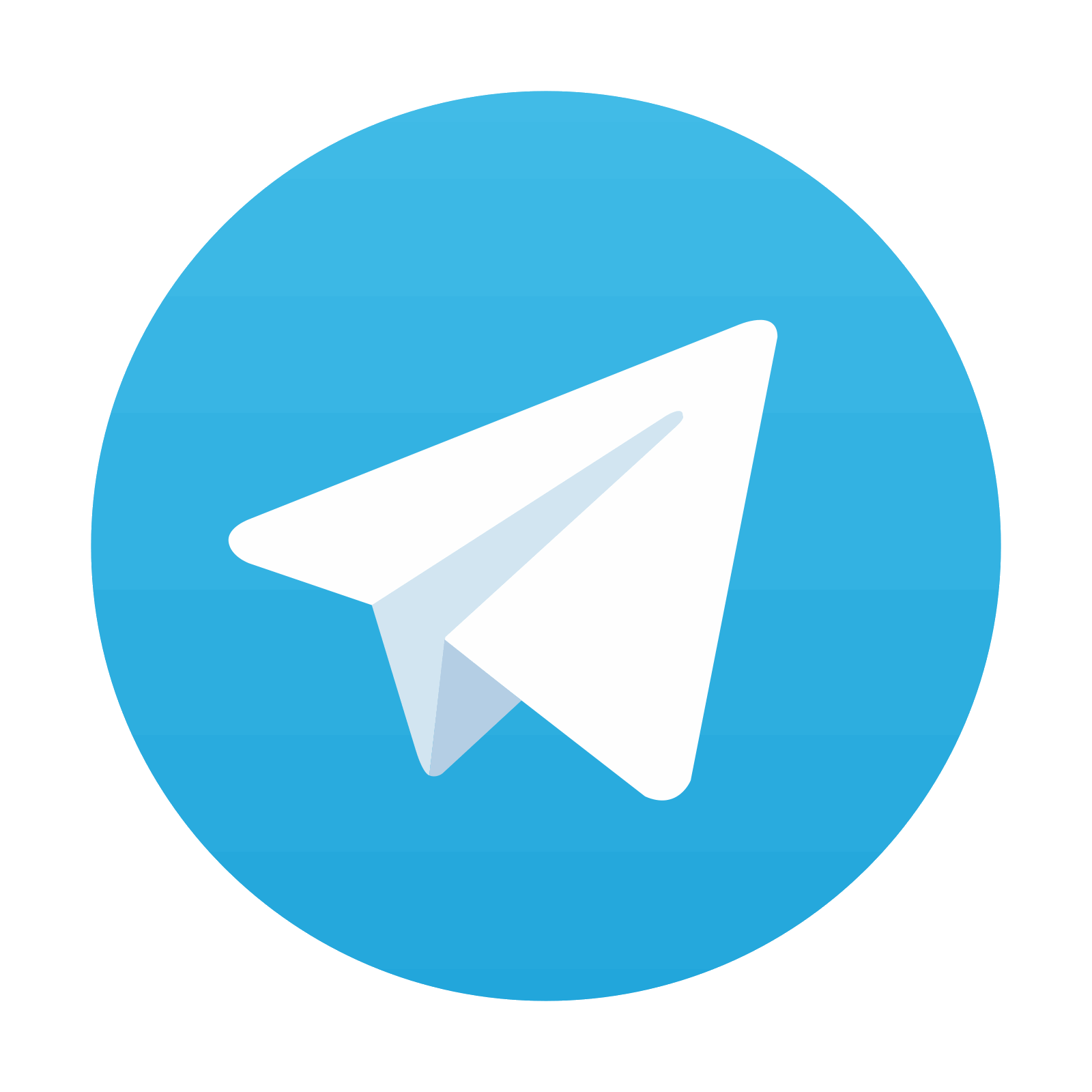
Stay updated, free articles. Join our Telegram channel
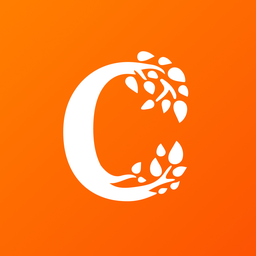
Full access? Get Clinical Tree
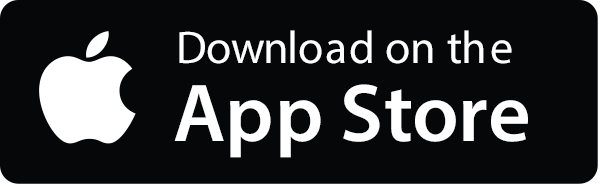
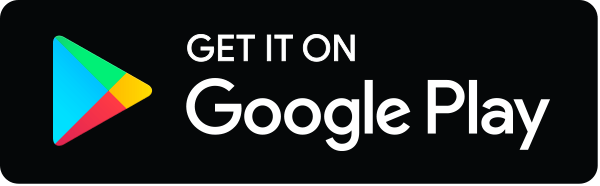