Fig. 1
Concept behind PALM. When labeled with a fluorescent probe and viewed through a conventional microscope, high spatial frequency detail in an object (leftmost column) is obscured, producing a diffraction-limited image (middle left column). PALM may be used to obtain a much higher-resolution image, if two criteria are met. First, the sample must be labeled with a photoswitchable probe and only a sparse subset of probes induced to activate and fluoresce in any given frame (middle right column). This “isolation” procedure alone is insufficient, as combining the recorded fluorescence from all N frames recovers the diffraction-limited image. However, if the optically resolved images of the individual molecules are also localized (rightmost column) with high precision, the aggregate image resulting from all such localizations may be combined to obtain a super-resolution image
Broadly speaking, photoswitchable probes may be divided into two broad categories: those that may be genetically expressed (e.g., photoactivatable fluorescent proteins, PA-FPs [5]) and those synthetic dyes that may be exogenously introduced via antibodies or small-molecule labeling strategies [6]. Each class has its own strengths and weaknesses: PA-FPs are small (a few nm in size), may be targeted with molecular precision and are thus highly specific, and may possess a high (>1,000) contrast ratio between bright and dark states, helpful in isolating a single fluorescent molecule from its neighbors and acquiring high-density (and thus high resolution) images. Unfortunately, PA-FPs are in general dimmer than most synthetic dyes, limiting localization precision and challenging the detection of activated single-molecule signal above cellular autofluorescence and residual faint background emission from the potentially large number of inactive molecules. They are also available only in limited colors, and the brightness and contrast ratio of the green-emitting PA-FPs is generally lower than the red-emitting PA-FPs (although the palette of PA-FPs continues to expand [7] and the performance of green PA-FPs continues to improve [8]). Switchable synthetic dyes can be very bright and are available in many colors [9], significant advantages over PA-FPs. However, the coupling method (e.g., to antibodies) can introduce significant uncertainty in their position, thus defeating the brightness advantage and effectively limiting resolution. Exogenously introduced dyes often label their target less specifically than PA-FPs, leading to an increased level of background in the reconstructed super-resolution image and sometimes introducing uncertainty as to whether localizations are real or spurious. Finally, many exogenous dyes require an additional chemical cocktail (typically reducing agents or oxygen scavengers) and/or high excitation intensities, limiting application in live cells. In this chapter, we focus on instrumentation particularly suited to the somewhat dimmer PA-FPs.
Initial PALM experiments were conducted in 2D [2, 10] near the coverslip surface, where optical aberrations and scattering are minimized, and the full numerical aperture (NA) of oil objectives may be used to maximize signal collection and aid in the localization precision of dim PA-FPs. An additional advantage of 2D imaging near the coverslip is that total internal reflection (TIR [11]) may be used to limit excitation to the focal plane and further aid in single-molecule detection. As it forms the basis for 3D experiments, we cover 2D PALM instrumentation in Subheading 3.1.
While suited to membrane [12] and focal adhesion [13] studies in live cells, the obvious drawback of TIRF is that the vast majority of the cell is inaccessible. The simplest way around this problem is to move the excitation out of TIRF, thus employing epi- or wide-field imaging to illuminate the entire cell. This imaging modality also creates several problems for 3D super-resolution imaging: (a) in addition to x and y, a molecule’s z coordinate must be localized with subdiffractive precision, and the resulting information used in assembling a 3D PALM dataset; (b) axial drift must be measured and/or controlled; and (c) background photoactivation and subsequent fluorescence of probe molecules at axial locations other than the focal (imaging) plane must be minimized, lest their localizations be wasted and the extraneous background signal so created impede localization in the focal plane. We discuss technical solutions to (a–b) in Subheading 3.2 and (c) in Subheading 3.3. We also refer to the reader to [14, 15], as this chapter draws heavily from these sources.
2 Materials
2.1 2D PALM Instrumentation
1.
IX-81 microscope frame equipped with TIRF illuminator, left-side port, DIC optics, filter cube turret, and 1.6× internal beam expander (Olympus America, IX2-RFACB2-R).
2.
Automated XY stage (Applied Scientific Instrumentation, MS-2000).
3.
High numerical aperture (1.49 NA) 60× objective lens, suitable for TIRF (Olympus America, APON 60XOTIRF).
4.
Filters in microscope frame, used for PA-FP imaging:
(a)
mEos2/pa-mCherry1 dichroic mirror (Semrock, FF562-Di02-25×36); bandpass.
(b)
mEos2/pa-mCherry1 emission filter (Semrock, FF01-617/73-25).
(c)
Dronpa/PS-CFP2 dichroic mirror (Chroma, T495lp).
(d)
Dronpa/PS-CFP2 bandpass emission filter (Chroma, ET525/50).
5.
Optical table (Technical Manufacturing Corporation).
6.
Overhead shelf system (Newport, ATS-10).
7.
Sticky pads to remove dust (Cole Static Control).
8.
Temperature/humidity USB monitor (Practical Design Group LLC, THUM).
9.
Excitation optics sled, optomechanics, and spacers for raising sled to TIRF port height (Thorlabs, PBH11106, BLP01, PF175, PS1, PS2, PS3, PS4).
10.
Lasers for common PA-FPS:
(a)
Excitation for mEos2, pa-mCherry1, 200 mW, 561 nm (Crystalaser, CL561-200).
(b)
Excitation for Dronpa/PS-CFP2, 150 mW, 488 nm (Newport, Cyan scientific laser).
(c)
Photoactivation of PA-FPs, 100 mW, 405 nm (Coherent, 405-100 CIRCULAR).
11.
Sled optics:
(a)
561 nm beam expansion, 2.7×, Thorlabs f = 15 mm LA1540-A and f = 40 mm LA1304-A.
(b)
488 nm beam expansion, 4×, Thorlabs f = 25 mm AC127-025-A-ML and f = 100 mm AC254-100-A-ML.
(c)
405 nm beam expansion, 2×, Thorlabs f = 15 mm LA1540-A and f = 30 mm LA1289-A.
(d)
Neutral density filters (Edmund Scientific, NT54-460).
(e)
Cage optomechanics for mounting beam expanders and neutral density filters (Thorlabs, CP02T, ER4, ER6).
(f)
Shutter, controller, and power supply (Thorlabs, SH05, TSC001, TCH002).
(g)
Visible mirrors and holders (Thorlabs, BB1-E02 and KS1).
(h)
External dichroic mirror for integrating 488 nm laser (Semrock, FF506-Di02-25×36).
(i)
External dichroic mirror for integrating 405 nm laser (Semrock, FF458-Di02-25×36).
(j)
Dichroic mirror holders (Thorlabs, KM100C).
(k)
Beam steering assembly (Thorlabs, GN05 and CVI/Melles Griot 12.5 mm diameter/5 mm thick fused silica windows).
(l)
Focusing lens (Edmund Optics, f = 50 mm achromatic lens, NT49-356-INK) and holder (Thorlabs, ST1XY-S and SM1ZM).
(m)
Collimating lens (Edmund Optics, f = 100 mm achromatic lens, NT49-360-INK) and holder (Thorlabs, ST1XY-S and SM1ZM).
(n)
Translation stage for imaging focused beam at back focal plane of microscope (Newport, 461-X-M and SM-06).
12.
Sample holder and stage insert (Applied Scientific Instrumentation, I-3033 and I-3033-25D).
13.
25 mm, #1.5 coverslips (Warner Instruments, CS-25R15).
14.
Back-thinned, electron-multiplying CCD (Andor Technology, DU-888E-C00-#BV).
15.
1.2× emission-side beam expander (Diagnostic Instruments, DD12BXC).
16.
Acquisition Computer (Advantech, SYS-4U-BTO with 2 TB hard drive, 2.5 GB RAM, Dual 2.13 GHz processors).
2.2 Additional Components for 3D PALM
1.
Automated XY translation stage with z piezo (Applied Scientific Instrumentation, PZ-2000).
2.
High numerical aperture (1.2 NA) 60× water-immersion objective lens (Olympus America, UPLSAPO60XW).
3.
Focus-lock system to minimize z drift (Mad City Labs, C-Focus).
4.
Gold fiducial particles, 100 nm (Microspheres-Nanospheres, 790122-010).
5.
Data analysis computer (Xi Computer Corp, 2P64 workstation with 500 GB hard drive, 8 GB RAM, 2× AMD Opteron processors 2 GHz, 8 cores).
6.
Network-attached storage device (Amazon.com, Netgear ReadyNAS + 2 TB internal hard drives).
7.
Cylindrical lenses for astigmatic localization (Thorlabs, f = 100 mm, LJ1567RM-A; f = 150 mm, LJ1629RM-A; f = 300 mm, LJ1558RM-A).
8.
Optomechanics for inserting cylindrical lenses between EM-CCD and microscope left-side port:
(a)
Filter wheel (Thorlabs, CFW6).
(b)
Adapter with external SM1 threads and internal C-mount threads (Thorlabs, SM1A10).
(c)
Adapters with (a) external C-mount threads and internal SM1 threads and (b) external SM1 threads (Thorlabs, SM1A9 and SM1T2).
(d)
1″ travel translation stage (Thorlabs, DT25).
(e)
Support for 1.2× expander (Thorlabs, RS2P, extra spacers as needed).
2.3 Components for Two-Photon Activation of PA-FPs
1.
Laser for two-photon activation (2PA), capable of producing pulses of 140 fs duration and tuned to 800 nm (Coherent, Chameleon Ultra II).
2.
Power control:
(a)
Glan-Laser calcite polarizer (Newport, 10GL08AR.16).
(b)
Half-wave plate for infrared wavelengths (Newport, 10RP52-2).
(c)
Motorized rotation mount (Thorlabs, PRM1Z8E).
(d)
Beam dump (Newport, PL15).
3.
Temporal focusing optics:
(a)
Periscope system for directing beam onto scanning mirror from above (Thorlabs, KCB1, CP02T, ER4, ER6, PH3, TR2).
(b)
Galvonometric scanner (Cambridge Technology, 6215HB).
(c)
Function generator for driving galvo (Stanford Research Instruments, DS340).
(d)
Focusing cylindrical lens (Thorlabs, LJ1653L2-B).
(e)
Cylindrical beam expander (Thorlabs, f = 30 mm, LJ1212L2-B, and f = 200 mm, LJ1653L1-B).
(f)
Low-dispersion silver mirrors, 1″ and 2″ diameter (Femtolasers, OA022 and OA248).
(g)
830-groove-per-millimeter gold-coated reflective diffraction grating with 21.4° blaze angle (Newport, 53107BK02-035R).
(h)
Magnetic mount for diffraction grating (Thorlabs, KB3X3).
(i)
Lens pairs for demagnifying grating onto sample (Thorlabs, f = 300 mm AC508-300-B-ML and f = 100 mm AC254-100-B-ML) and (Thorlabs, f = 500 mm AC508-500-B-ML and Olympus, UPLSAPO60XW f = 3 mm objective).
4.
Coupling optics for combining 800 nm light with visible wavelengths used in single-photon excitation:
(a)
Removable mirror mounted on a magnetic base (Thorlabs, BB1-E02 and KB1X1).
(b)
Visible achromatic lens, f = 400 mm (Edmund Optics, NT49-369-INK).
(c)
External dichroic mirror for combining visible and 800 nm illumination prior to microscope body (Semrock, Di01-R561-25×36).
(d)
Periscope unit for raising 800 nm illumination and directing it into the microscope frame (Thorlabs, KS2, RS4P, RS6, RS1, RM1C).
(e)
Right-side port attachment on microscope frame for illumination (Olympus, IX2-RFACB2-R).
(f)
Filter cube suitable for right-side port illumination (Olympus, IX2-MFB-SP-R).
(g)
Custom dichroic mirror to be placed inside filter cube (Chroma, ZT405/488/561/IR-RPC, reflects 405 nm, 488 nm, 561 nm, 700–1,100 nm).
(h)
Shortpass emission filter, for blocking 800 nm pump light, to be placed inside filter cube (Semrock, FF01-680/SP-25).
5.
Diagnostics for measuring quality of temporal focus:
(a)
Quantum dot solution (Q dots; Ocean NanoTech, QSO-520-0010).
(b)
Ethylene vinyl acetate copolymer resin (DuPont, Elvax 410).
(c)
Toluene (Sigma, 244511).
(d)
Glass vial (Fisher Scientific, 03-339-22C).
(e)
Hot plate (Corning, PC-220).
(f)
Spin coater (Laurell Technologies Corporation, WS-650S-8NPP-LITE).
3 Methods
3.1 2D PALM Instrumentation
As instrumentation for 2D PALM has been covered extensively elsewhere [15], here we review major concepts only. Much of the following description also applies to 3D PALM; exceptions for TIRF are noted when appropriate. Data acquisition and processing are covered in Subheading 3.2.
3.1.1 Basic Considerations
PALM instrumentation should be housed in a clean, dust-free environment to reduce sample contamination and avoid the stray light and scattering caused by dust in the optical path. We place sticky pads outside the room housing the PALM to help reduce dirt brought in by users. While the undesirable effects of thermal drift may be compensated for by post-processing (Subheading 3.2.4), it is advantageous to control the temperature as much as possible to mitigate this problem. We periodically monitor the temperature using a USB device in order to ensure temperature is within specification. To reduce mechanical vibrations, we bolt all optics on a vibrationally isolated air table (see Note 2 ).
3.1.2 System Overview
Although it is possible to build a PALM system entirely from “the ground up” [2], we find it helpful to use a commercial microscope frame (Olympus, IX-81) as the base because the eyepieces, bright-field optics, objective holder, and filter cube turret make it easy to both screen samples and examine them with additional, non-PALM imaging modes (e.g., differential interference contrast (DIC)). Another advantage of buying a commercial frame is that the TIRF illuminator add-on facilitates TIRF by enabling the user to free-space couple high-intensity lasers of the appropriate wavelength into the system, thus minimizing PALM acquisition time. Also, the microscope manufacturer can provide an automated XY stage (e.g., ASI, MS-2000), helpful in scouting for appropriate samples and in marking their positions. With the frame bolted to the optical table, the main additional components are the excitation and activation optics, the optics housed within the microscope frame itself, and the detection optics (Fig. 2a).
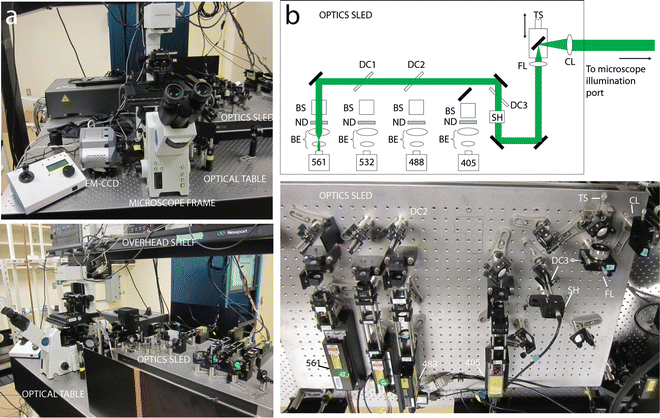
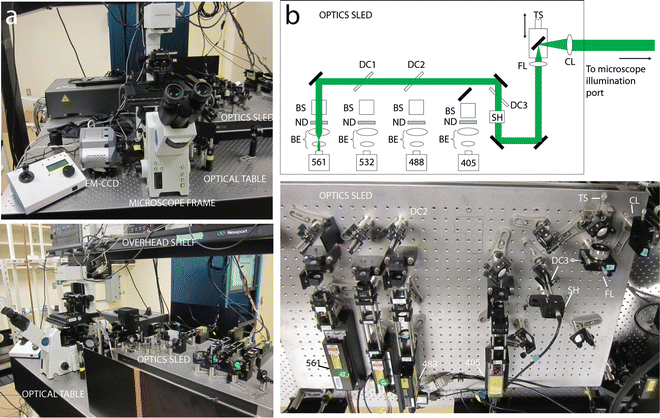
Fig. 2
Basic (2D) PALM system. (a) Front (top) and side (bottom) views, showing major items referred to in text including microscope frame, vibration isolation table, overhead shelf, and excitation sled. (b) Expanded schematic (top) and photograph (bottom) of excitation/activation lasers, associated optics, and optomechanics. Symbol key: DC dichroic mirror, BS beam-steering assembly, ND neutral density filter, BE beam expander, SH shutter, TS translation stage, FL focusing lens, CL collimating lens. Lasers are labeled according to wavelength, as referred to in the text. Lenses are drawn as ellipses in the schematic, reflective mirrors as filled–in rectangles. Note that DC1 and the 532 nm laser are not used for exciting PA-FPs and are thus not referenced in the text. Panel (b) is modified from York 2011, supplementary Fig. 1 with permission from Nature Methods
3.1.3 Excitation and Photoactivation
As almost all PA-FPs are photoactivated with near-ultraviolet light, we use the commonly available 405 nm laser for PALM (Coherent, 405-100 CIRCULAR). Red PA-FPs (pa-mCherry1 or mEos2) are efficiently excited with a 561 nm laser (Crystalaser, CL561-200); for green PA-FPs (Dronpa or PS-CFP2), we use a 488 nm laser (Newport, Cyan scientific laser). We bolt all lasers to a small optical sled (Thorlabs, PBH11106), beam expand each to a common diameter, and combine their output with dichroic mirrors (Fig. 2b). We introduce beam-steering assemblies in front of each laser to correct for small differences in laser height or lateral position. Cage-mount optomechanics (Thorlabs, CP02T, ER4, ER6) are particularly useful in mounting beam expanders or neutral density filters and assure colinearity of optics along the beam path. Finally, we place a computer-controlled, mechanical shutter (Thorlabs, SH05, TSC001, and TCH002) after the coaligned beams, allowing the user to easily turn off the illumination to the sample.
3.1.4 Achieving TIRF
As discussed elsewhere [15], TIRF is achieved by focusing the coaligned beams at the edge of the back focal plane (BFP) of the objective lens. We focus the laser illumination on a sled with lens FL and recollimate the beam with lens CL. By positioning the entire laser system such that the back focal plane of lens CL is coincident with the front focal plane of the 200 mm relay lens internal to the TIRF illuminator (see Note 3 ), the focus created by FL is imaged at the BFP. In this geometry, the image of the focus can be moved at the BFP by translating a mirror placed on translation stage TS. The “U-DP” attachment on the TIRF illuminator is useful for switching between arc lamp and TIRF illumination, but only the 200 mm relay lens within the illuminator is necessary; the entire fiber-coupling assembly can be removed.
3.1.5 Microscope Body and Sample Holder
Dichroics and emission filters suitable for green or red PA-FPs can be placed directly into commercially available filter cubes and placed into the filter turret assembly (see Note 4 ). Most PALM samples are cells that may be cultured directly on glass coverslips. We use 25 mm round coverslips (Warner Scientific) and place them in a round coverslip holder (ASI, I-3033-25D). The holder and coverslip may be placed inside an insert (ASI, I-3033) that can be secured on the automated stage.
3.1.6 Detection Optics and Camera
For optimal single-molecule localization, the pixel size of the detector must properly sample the point-spread function [16]. Given the pixel sizes of most cameras, this usually implies additional magnification besides the magnification provided by the objective lens. With a 60× objective, we use the 1.6× internal magnifier housed within the microscope frame and a 1.2× external lens, thus providing a total magnification of 115.2 between sample and camera.
We currently employ a back-thinned EM-CCD (Andor, DU-888E-C00-#BV, see Note 5 ) with 13 μm pixels, for a final imaging pixel size of 113 nm.
3.2 3D PALM
With relatively few additional components, the microscope described above can be converted to a 3D PALM system capable of sub-100 nm resolution in all three dimensions. Major additional requirements include a water-immersion objective better suited for 3D samples, minimization and compensation of drift during the acquisition period, cylindrical optics for converting a molecule’s axial position into a PSF shape change, and data processing software capable of processing the raw data into a list of 3D coordinates and rendering the coordinates into a PALM image.
3.2.1 Water-Immersion Objective
For 3D samples that are immersed in aqueous buffer and that are more than a few 100 nm thick, water-immersion objectives are better suited for PALM than the oil objectives used in TIRF. This is for several reasons: (a) the refractive index of most cellular samples is between 1.33 and 1.38; thus, an objective with greater NA does not realize its full resolution potential or collection efficiency. (b) Spherical aberration (due to refractive index mismatch between the oil immersion media and the aqueous sample) causes an oil objective’s performance to degrade at increasing depths from the coverslip. The resulting broadening of the PSF (especially axially) spreads the available signal out, decreasing the signal-to-noise ratio (SNR) per pixel in the raw images. This is especially problematic when localizing dim PA-FPs, where signal is precious and a significant decrease lowers the localization precision or renders the molecule undetectable. At the macroscopic level, the “focal shift” introduced by the refractive index mismatch introduces undesirable axial image distortion. Such deleterious effects may be partially compensated for by adding index-matching reagents to the sample (e.g., glycerol) or by scaling the axial localizations by the ratio of refractive indices [17]. We find it simpler to use a water-immersion objective (Olympus America, UPLSAPO60XW), matching the refractive index of our fixed cellular samples (see Note 6 ) and avoiding these effects while simultaneously maintaining a relatively high NA (1.2) (see Note 7 ). This objective also has good infrared transmission, important for activating PA-FPs with 800 nm light (Subheading 3.3).
3.2.2 Piezoelectric z Stage and Axial Drift Reduction
To assemble a “z stack” or collection of imaging planes that span a 3D sample, it is necessary to move the sample relative to the imaging plane. We use an automated XY stage with an additional z piezo upgrade that permits 100 μm axial displacement of the sample (Applied Scientific Instrumentation, PZ-2000). This replaces the 2D stage in Subheading 3.1.
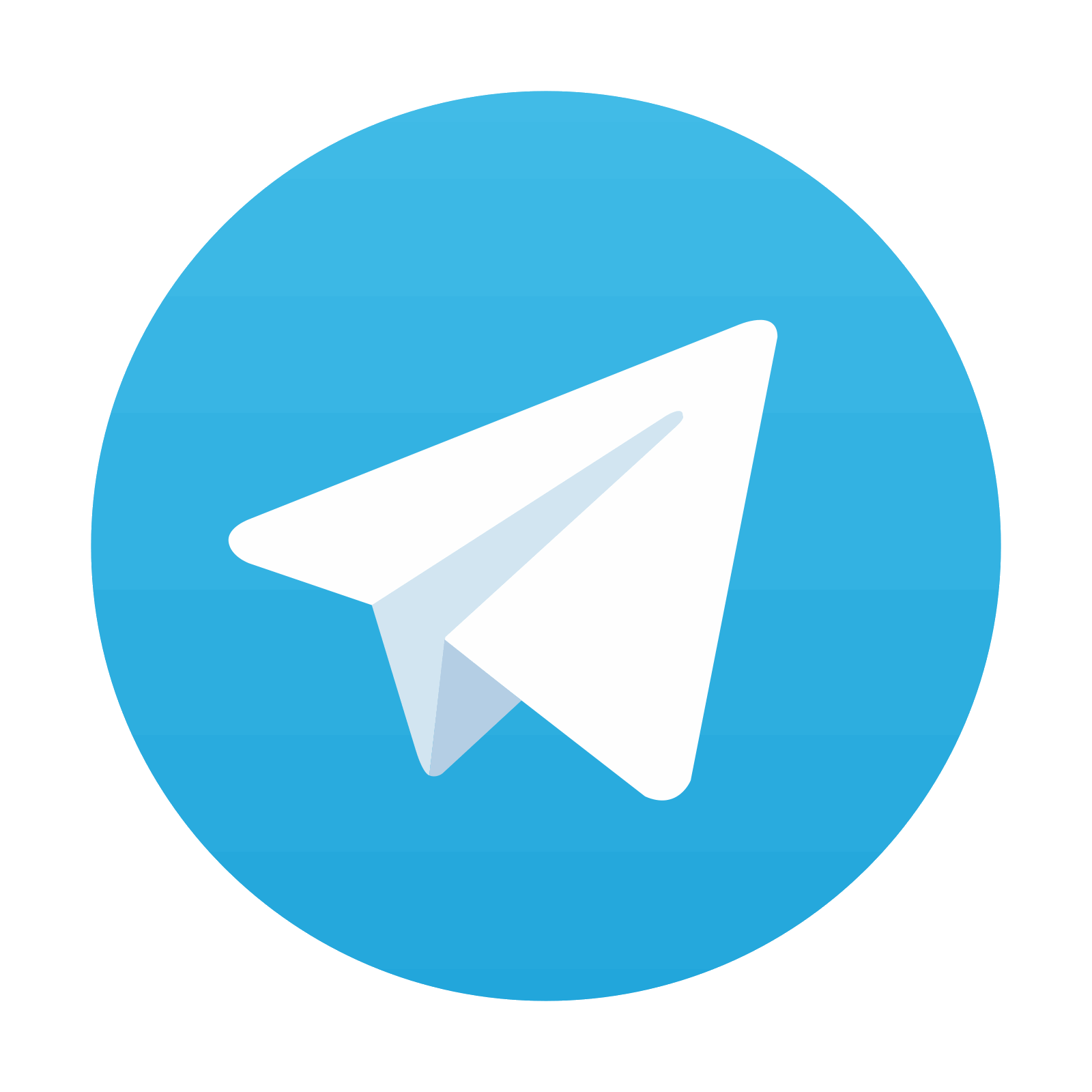
Stay updated, free articles. Join our Telegram channel
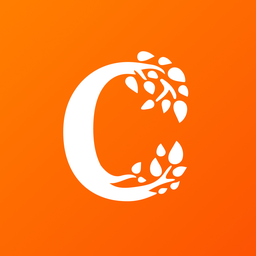
Full access? Get Clinical Tree
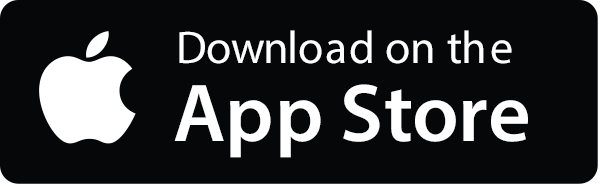
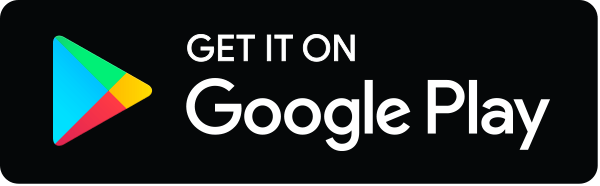