Fig. 21.1
Nodular malignant melanoma . Cutaneous melanoma of the nodular subtype. Please note the red halo around the lesion representing an inflammatory immune response against neo-antigens present in the tumor (with permission from © 2016 BMJ Publishing Group Ltd. All rights reserved)
Embryology and Phenotypic Heterogeneity
To better understand the behavior of melanoma cells in host tissues, it is necessary to consider the normal developmental biology of the melanocyte. Many of the features of melanoma cell behavior can also be observed during melanocyte development, with the exception that the latter is under a strict control and fine-tuned during evolution. For melanoma cells, these features include returning to a proliferative, dedifferentiated, and migratory phenotype similar to is observed during epithelial-to-mesenchymal transition (EMT) in epithelial cancers. In fact, many of the same genes involved in melanoma progression and metastasis are active during normal development (Table 21.1).
Table 21.1
A selection of genes involved both in normal melanocyte development and melanoma development [147]
Gene | Function | Specification | Migration | Proliferation | Survival | Differentiation | Melanoma |
---|---|---|---|---|---|---|---|
PAX3 | Transcription factor | X | X | X | X | ||
LEF1 | Transcription factor | X | X | X | |||
SNAI2/SLUG | Transcription factor | X | X | X | |||
SOX9 | Transcription factor | X | X | X | X | ||
WNT1 | Wnt signaling | X | X | X | X | ||
β-catenin | Intracellular signaling, transcription | X | X | X | |||
Cadherins | Surface binding proteins | X | X | ||||
Integrins, laminin, fibronectin | Extracellular matrix proteins | X | X | ||||
SOX10 | Transcription factor | X | X | X | X | X | X |
MITF | Transcription factor | X | X | X | X | X | X |
KIT | Tyrosine kinase | X | X | X | X | X | X |
SCF | Stem cell factor, ligand for c-KIT | X | X | X | X | X | X |
FGFR | Fibroblast growth factor receptor | X | X | X | |||
bFGF | Basic fibroblast growth factor | X | X | X | |||
MC1R | G protein receptor | X | X | X | |||
BCL-2 | Apoptotic protein | X | X | ||||
MET | Tyrosine kinase | X | X | X | X | ||
HGF | Hepatocyte growth factor. C-met ligand | X | X | X | X |
During embryonic development, the melanocyte originates from pluripotent cells in the dorsal edge of the neural tube and further differentiates in the neural crest. These stem cells have the ability to differentiate into a variety of specialized cells like neurons, glial cells, cardiac cells, as well as pigment cells [1]. The developing melanocyte will undergo four important steps before it arrives at the final destination and differentiates into mature melanocytes: specification, migration, survival, and proliferation. These four stages are regulated by genetic programs defined by specific sets of genes outlined in this section (for a detailed review, see [2]). During early gestation, cells destined to become melanoblasts begin expressing genes encoding transcription factors that further push the cells toward differentiation into specialized pigment cells. Of special importance are PAX3, LEF1, FOXD3, as well as genes involved in the Wnt signaling pathway [3–7]. In addition, the transcription factors SOX9 and SNAI2/Slug play a central role in initiating EMT and in activating MITF, thereby preparing the melanoblast for migration [8, 9]. Microphthalmia-associated transcription factor (MITF) is regarded as the master regulator of melanocyte differentiation [10, 11] and is frequently amplified or overexpressed in melanoma [11–13]. In addition to the melanoblast intrinsic expression pattern of genes, paracrine factors produced in the local environment are also involved in regulating the migrating melanoblast. For example, stem cell factor (SCF) is able to regulate gene expression programs, like MITF expression, through interaction with its cell surface receptor c-Kit and the downstream MAP kinase pathway [14]. Also, the cell-cell adhesion molecule β-catenin has been shown to activate MITF [15]. Cell-cell and cell-matrix interactions are also important for the migrating melanoblast. The melanoblasts need to escape the tissue of origin (neural crest) and not get stuck in any structures before the destination is reached. Up- and downregulation of various proteins, including cadherins, ensure the appropriate interaction with components of the extracellular matrix (ECM) , such as integrins, laminin, lectins, and fibronectin [16, 17]. The melanoblasts also need to force themselves through biologic barriers and basement membranes to get to their destination tissues, for instance, by upregulating the expression of metalloproteinases like ADAMTS20 [18].
Interestingly, melanoblasts proliferate extensively before and after their migration but are more quiescent during the migration phase [19], again similar to the EMT process in epithelial tumors. This points to the importance of cellular plasticity and phenotypic switching in melanocytes, as well as in their malignant counterparts, melanoma cells. The phenotype-switching model of melanoma postulates that melanomas switch between proliferation and invasion (“growing or going”), but they rarely do both simultaneously [20, 21]. As BRAF inhibition and chemotherapy attack melanoma cells during the proliferative state, there will always be a subset of cells in a nonproliferative state that are less sensitive to these drugs [7]. There is even data to suggest that these migrating cells are evading an immune attack [22, 23]. In melanoma, Snail-induced EMT accelerates cancer metastasis through both enhanced invasion and induction of immunosuppression due to induction of regulatory T cells, immunosuppressive cytokines, impaired dendritic cells, and direct cytotoxic T-lymphocyte resistance. EMT-reversal by Snail blockade simultaneously inhibited both cancer invasion and multiple immunosuppressive mechanisms, resulting in efficient inhibition of cancer metastasis [24].
Considering the relative small size of primary tumors at the time metastases are observed, no other cancers can compete with the melanoma in eagerness to invade and metastasize. This aggressive feature of melanomas does in part rely on their ability to rapidly switch between phenotypes. The significant cellular plasticity observed in melanoma is reflected in the switching between a differentiated and an invasive phenotype, under the control of MITF and several different EMT-associated transcription factors [10]. Phenotypic heterogeneity is a result of (epi)genetic changes as well as of changing conditions in the tumor microenvironment. Growth factors and transcription factors inducing a more mesenchymal-like phenotype, i.e., motile and invasive, like TGFβ, HIF1α, ZEB1, SNAIL, and TWIST, are upregulated under stressful conditions such as hypoxia, UV-radiation, and inflammation [25–27]. These stress-responsive cellular programs are beneficial for melanoma cells under harsh conditions in hostile environments like the blood stream or other refractory tissues. Cellular stress responses like hypoxia have been extensively studied in melanoma. In a study by O’Connel and coworkers, an adaptive phenotype shift was described in response to hypoxia in the tumor microenvironment [7]. A rapid shift in expression, between Wnt5A receptors ROR1 and ROR2, was observed in response to hypoxia. Hypoxia thus induced a switch from a proliferative ROR1-positive phenotype to a more invasive ROR2-expressing phenotype. Of significant importance, this switch led to a tenfold decrease in sensitivity to BRAF-inhibitors in clinical use.
Going back to normal melanocyte development, once the migration is completed and the melanocyte arrives at the intended destination, another cellular program takes over. To ensure proliferation, maturation, and survival, the melanocyte relies on growth factors such as basic fibroblast growth factor (bFGF) and hepatocyte growth factor (HGF) to support the final steps in their development into functional melanocytes, i.e., in the dermal-epidermal junction of the skin [28].
The close relationship between melanocytes and the basal keratinocytes, which they protect from the damaging UV-light of the sun, is both quiet and balanced. These cells (basal keratinocytes and melanocytes) are highly stable. Similarly to the malignant counterpart, once the melanoma cell has survived the journey from the primary to a distant site, regrowth, differentiation, and escape from a micrometastatic state, is initiated by regaining MITF, SLUG, and ZEB2 [10, 11, 29]. The balance between the stem cell-like, differentiated, and invasive melanoma phenotype is illustrated in Fig. 21.2 (from [10]).


Fig. 21.2
Epithelial-to-mesenchymal transition (EMT) inducing transcription factors in physiological and pathological development of the melanocyte lineage. EMT-inducing transcription factors regulate stemness and differentiation in melanocytes (left), whereas they determine the oscillation between differentiated vs. invasive cancer cells in melanoma (right). Phenotype switching that accounts for melanoma heterogeneity depends on a signaling switch of different EMT-inducing transcription factors and is regulated by microenvironmental cues, (epi)genetic instability, and oncogenic signaling (reproduced with permission from Vandamme & Berx (11))
Taken together, the processes at work during melanocyte differentiation, migration, and colonization can be also observed when the cell becomes malignant. Both the genetic programs controlling these processes and the interactions with the neighboring cells and matrix play a decisive role during progression. We have learned that the malignant melanoma cell can reactivate and co-opt the programs necessary for melanocyte development and thereby travel to distant organs. With this in mind, the next paragraphs will review the supporting and inhibiting interactions at play between melanoma cells and their microenvironment.
Interactions with Other Cells and Tissues One by One
Keratinocytes and the Skin
Normal melanocytes reside at the dermal-epidermal junction of the skin between basal keratinocytes and directly on top of the basement membrane. Their role is to produce melanosomes. Packed with melanin and through a complex network of dendrites, melanosomes are distributed among the adjacent keratinocytes and, once in place, protecting them from damage, e.g., by UV-light, from the external environment. The stability of this intimate relationship between melanocytes and keratinocytes is ensured through cell-cell adhesion molecules and paracrine growth factors. A well-functioning “melanin unit” (melanocyte and basal keratinocyte) is necessary to create a sanctuary for the skin cells living under otherwise stressful conditions. The most important known cause of melanoma is the damaging effects of UV-light. UV-light can induce specific mutations driving oncogenesis [30], but UV-light can also directly disturb the fine interactions between keratinocytes and melanocytes, reducing the antiproliferative and anti-invasive influence from keratinocytes on melanocytes [31]. This critical homeostasis between pigment cells and their microenvironment is disrupted during melanoma initiation. Following genomic damage, oncogene overexpression, and subsequent reprogramming of the melanocyte, the melanocyte escapes the control previously imposed on it by keratinocytes and initiates independent growth [31].
A significant part of the current knowledge about melanoma biology and the role of the complex microenvironment is the result of work performed by Dr. Meenhard Herlyn and his team at the Wistar Institute in Philadelphia [32]. Since most basic research on melanoma cells was performed on isolated cells growing in monocultures on plastic, an artificial condition that does not factor in the importance of an appropriate microenvironment, new and more sophisticated experimental models were needed. Three-dimensional organotypic cultures and collagen-implanted spheroids now offer new insight on how melanoma cells behave and respond to physiological stress as well as applied drugs in a more relevant setting, reflecting the behavior of melanoma cells in patients [33].
Upon malignant transformation, melanoma cells escape the control imposed by the keratinocyte by downregulation of cell adhesion molecules like E-cadherin, P-cadherin, desmoglein, and connexins [17, 34]. As illustrated in Fig. 21.3, the extracellular regions of cadherins create a homotypic interaction with cadherins on neighboring cells. The cytoplasmic domain of these transmembrane molecules is linked to the cytoskeleton and interacts with protein complexes including β-catenin. As we know from embryology, EMT, and stress responses described above, E-cadherin is downregulated, and N-cadherin is upregulated, making melanoma cells capable of interacting with other N-cadherin-expressing cells, like endothelial cells and fibroblasts, residing in the dermis. This critically important cadherin switch is one of the earliest steps in the creation of the invasive vertical growth phase of melanoma. Loss of E-cadherin expression, as part of resetting the cellular program to an invasive and stress-responding phenotype, also results in reduced inhibition of β-catenin signaling including upregulation of stress response genes such as c-Myc, cyclin D1, and MITF [35, 36] and silencing of the p16 tumor suppressor gene [37]. Loss of this specific inhibition will, in turn, stimulate the melanoma cells to proliferate. On the other hand, increased survival of transformed melanoma cells is supported by repression of proapoptotic factors like Bad as a consequence of increased expression of N-cadherin [38]. Basically, these tumor-promoting processes are not only independent changes in individual proteins happening by chance in a genetically unstable and chaotic tumor cell but rather a dramatic result of a cellular program with striking similarities to normal biological processes, such as stress responses, wound healing, and embryonic development.


Fig. 21.3
Keratinocytes and melanoma cells. The extracellular regions of cadherins create a homotypic interaction with cadherins on neighboring cells. During melanoma development, E-cadherin is downregulated and N-cadherin is upregulated, making melanoma cells capable of interacting with other N-cadherin-expressing cells, like endothelial cells and fibroblasts, residing in the dermis. This critically important cadherin switch is one of the earliest steps in the creation of the invasive vertical growth phase of melanoma
Although little is known of what controls the cadherin switch, the evidence points to an alteration in the cellular programming discussed above. It has been shown that the EMT inducers Slug and Snail inhibit E-cadherin at the transcriptional level [39, 40]. Li and coworkers showed that autocrine HGF decouples melanomas from keratinocytes by downregulating E-cadherin and desmoglein 1 [41], indicating an important role of C-met/HGF. In turn, this interaction is counteracted by the activation of the semaphorin 4D/Plexin B1 ligand/receptor complex, which again is downregulated by UV-light [42, 43]. Interestingly, Plexin B1 is a downregulated, downstream target of the frequently activated B-Raf/Mek/ERK pathway and seems to have a tumor-suppressing function in early melanoma development [44]. Taken together, this illustrates how the reprogrammed transformed melanoma cell can actively influence its relationship with the ECM and thereby increase its likelihood of succeeding in invading and metastasizing. As a net outcome, the cadherin switch ensures a program change in the transformed melanoma cell from a dormant, nonproliferating cell under strict control by the keratinocyte into an invasive, proliferating, apoptosis-resistant melanoma cell able to escape from the site of origin and to metastasize.
Whereas the cadherins ensure appropriate cell-cell adherence, the integrins anchor the melanocytes to the ECM (Fig. 21.3). In addition, the integrins are involved in controlling proliferation, invasion, immune response, angiogenesis, and survival [45]. Integrins are transmembrane heterodimers composed of one α- and one β-subunit. Binding of a ligand to the extracellular domain leads to a conformational change inducing signaling cascades in the cells, such as MAPK, PI3K, and NFκB. Importantly, conformational changes in the extracellular region of integrins can also be induced by the binding of signaling molecules to the intracellular part, thus leading to changes in how the cell responds and interacts with the microenvironment [32]. Integrins play an important role in modulating the transformation of signals mediated by various growth factor receptors in response to ligation of growth factors produced in the microenvironment. Receptor tyrosine kinases like epidermal growth factor receptor (EGFR), vascular endothelial growth factor receptors (VEGFRs), platelet-derived growth factor receptor (PDGFR), and focal adhesion kinase (FAK) [32, 45, 46] have major influence over the ability of tumor cell to proliferate, migrate, invade, and survive. Thus, the activity and composition of the integrin apparatus in the cell have an important role in modulating these processes. Especially important for the transition from horizontal to vertical growth of melanoma cells is the αvβ3 integrin. Increased expression of this specific transmembrane heterodimer results in increased production of the antiapoptotic factor bcl-2 as well as ECM degrading proteins like MMP-2 [47–49].
The melanoma cell has now escaped the suppressing influence of the keratinocyte and entered the vertical growth phase. There, it will encounter cells that its ancestors, the melanocytes, have not interacted with since the time of migration from the neural crest to the skin during embryonal development. These interactions can be supportive or suppressive depending on how the new host tissue responds to the intruder.
Seed, Soil, and Melanoma Stem Cells
More than a century ago, after studying 735 cases of fatal breast cancer, the surgeon Stephen Paget formulated his famous “seed and soil” hypothesis. He observed that some environments were more receptive for metastatic growth than others and concluded that secondary tumor growth at distant sites is both dependent on properties of the “seed” (cancer cell) and of the “soil” (microenvironment) [50]. Acknowledging the properties of the melanocyte during embryonic development to migrate, invade, and colonize distant organs (i.e., skin, eye, mucosa), it is of no surprise that circulating melanoma cell can find fertile soil and establish metastases at almost any site.
The acquisition of more mesenchymal-like attributes together with the downregulation of epithelial traits during epithelial-to-mesenchymal transition (EMT) and the reversion of this process, mesenchymal-to-epithelial transition (MET), is important for the metastatic process [51]. Progenitor cells, cancer stem cells, and metastatic cells can be “seeds” evolved from the primary tumor that may establish metastasis in proper niches or organ microenvironments (“soil”) [52].
Melanomas metastasize mainly through the lymphatic system but also via hematogenic dissemination to more distant sites like the lungs, liver, and brain. The metastatic process includes local invasion into blood vessels or lymphatic channels, transportation of multicell aggregates to distant sites, arrest in the capillary bed and subsequent extravasation into organ parenchyma, and, finally, establishment in the new microenvironment and further proliferation and tumor expansion [52]. These processes can be interrupted by the antitumor host response at many different levels and are therefore fully completed only by a very limited number of cells. Nowell suggested that acquired genetic variability during tumor progression together with selection pressure results in more aggressive tumor cells [53]. This concept of clonal origin of metastases has been verified by others and may explain heterogeneity in response to chemotherapy [52].
The role of cancer stem cells (CSCs) in the pathogenesis of melanoma metastasis is still under investigation, but there is evidence for an association between the presence of CSCs and disease progression, increased potential for metastases, and worse prognosis [54]. CSCs in melanoma are also named malignant melanoma-initiating cells (MMICs) [54]. CSCs are defined by three characteristics: the ability to initiate tumor growth, the capacity of self-renewal, and the ability to differentiate into tumor cells. ABCB5 and CD271 are markers characterizing MMICs in melanoma; others are under investigation. Increased expression of CD271 which promotes immune evasion and expression of ABCB5 is a characteristic in chemo-resistant cells. Furthermore, MMICs may promote invasion and metastasis in part reflected by EMT and MET [54]. Other melanoma stem cell markers are CD20, CD133, and ABCG2 [54].
Fibroblasts and Connective Tissue
During tumor progression and invasion, the transformed melanoma cells secrete growth factors to modulate the new microenvironment in a paracrine manner and to stimulate proliferation and survival in an autocrine manner. One important growth factor is basic fibroblast growth factor (bFGF) , and most melanomas produce and secrete bFGF [55, 56]. Whereas the normal melanocyte does not produce bFGF and relies on paracrine bFGF from fibroblasts and keratinocytes, melanoma cells can co-express bFGF and the FGF receptor [55–57]. Interestingly, in both melanomas and melanocytic nevi, the expression of bFGF seems to be highest in the dermal tumor areas [55]. Most cells in the tumor microenvironment are able to express and secrete bFGF and bFGF with different consequences. In addition to increased proliferation and survival of melanoma cells [58], bFGF secretion by invading melanoma cells stimulates growth of endothelial cells and fibroblasts and consequently promotes angiogenesis and fibrous stroma formation [59].
The fibroblasts in the tumor microenvironment are also stimulated by platelet-derived growth factor (PDGF) secreted by the transformed melanoma cells. In response to PDGF, the fibroblasts produce ECM proteins, such as collagen, fibronectin, and laminin [60], as well as growth factors like bFGF, IGF-1, and TGF-β [32]. These ECM proteins convert the neoplasm from a soft mass into a firm tumor. Similarly, paracrine secretion of transforming growth factor beta (TGF-β) by melanomas also has a multitude of consequences in the tumor microenvironment, including increased deposition of ECM proteins, angiogenesis, and immunosuppression [61].
Normal dermal fibroblasts suppress growth and progression of premalignant melanoma lesions at early stages [62]. In part, normal dermal fibroblasts exert this effect by mobilizing immune cells via the secretion of cytokines, such as interferon γ, interleukin 6, and TNFα [63]. In addition, normally functioning dermal fibroblasts create a physical barrier for the melanoma cells. To penetrate the dense dermis, invading melanoma cells need to modulate the ECM and do this by expression of several different matrix metalloproteinases. In healthy tissue, normal dermal fibroblasts are able to regulate and restrain these changes, thereby preventing degradation of the basement membrane and blocking invasion (reviewed in [64]). In contrast, cancer-associated fibroblasts (CAFs) are believed to be heterogeneous with similar properties as the activated myofibroblasts frequently found under inflammatory conditions and wound healing. CAFs express α-smooth muscle actin (α-SMA), fibroblast specific protein-1 (FSP-1), fibroblast-activating protein (FAP), PDGF, and α11β1 integrin [64, 65]. CAFs in the tumor stroma originate from different sources, such as from bone marrow mesenchymal cells, EMT of resident epithelial cells, or by recruitment and activation of resident normal dermal fibroblasts [64, 66]. The interaction between melanoma cells, actively trying to break down the basement membrane, and fibroblasts residing in the dermis is probably the major cause of the recruitment of CAFs in the invading melanoma (Fig. 21.4).


Fig. 21.4
Melanoma-fibroblasts-CAFs . Initially, invading melanoma cells are inhibited by normal fibroblasts acting as a physical barrier in the dermal stroma. Melanoma cells can interact with the fibroblasts in the ECM by expressing several different factors, such as bFGF, PDGF, and TGF-β, and thereby contribute to the transformation of the dermal fibroblasts into cancer-associated fibroblasts (CAFs). Once CAFs are established around the invading tumor border, they further stimulate the progression of the melanoma cells
Once stimulated and established in the ECM, CAFs can promote tumor growth in different ways. Following the E-cadherin to N-cadherin shift in melanoma cells, CAFs can interact through direct cell-cell contacts, mediated through N-cadherin, and help melanoma cells to migrate away from the restraining effects of keratinocytes and invade into the underlying dermis. CAFs produce a number of ECM proteins, such as laminin and fibronectin, which are structural components that make up the connecting tissue and contribute to the dense fibrous nature of solid tumors [67]. In addition, CAFs are able to remodel the ECM through secretion of MMPs [68] and fibroblast activation protein(FAP)-α, thus facilitating further growth and migration by melanocytes. Hypoxic stress in the TME induces a stress response in melanoma cells, which in turn can send signals to the surrounding CAFs to secrete growth factors such as vascular endothelial growth factor (VEGF), stromal-derived factor-1 (SDF-1), IL-6, as well as a number of chemokines, thus favoring angiogenesis, chemotaxis, and invasion [64, 69]. Taken together, after leaving the suppressive partnership with the keratinocytes, the promiscuous melanoma cell now interact with CAFs as their new supporters, and together they can overcome any obstacle they meet in the dermis on the way to metastasizing.
Endothelial Cells and Vasculature
In 1971, Dr. Judah Folkman postulated that tumor growth beyond the size limit of about 1–2 mm, the maximum diffusion limit oxygen in tissue, is dependent on angiogenesis [70]. Angiogenesis is the establishment of new vessels by sprouting from preexisting vasculature. After the melanoma has entered the vertical growth phase and penetrated the basement membrane, angiogenesis is required for further tumor growth [71]. Without the capability to induce angiogenesis, the melanoma will linger on in dormancy as a microscopic lesion for years. Following an angiogenic switch [72, 73], the dormant tumor will enter a phase of rapid growth followed by metastasis, morbidity, and death. The triggering factor for the angiogenic switch is not yet completely identified and will be discussed later when discussing ulceration and wound healing.
As mentioned earlier, melanoma growth in a hostile site (local or metastatic) is associated with a tremendous stress on the melanoma cells. To survive such stressful conditions, stress response programs are initiated in the cell. Hypoxia is such a major stress factor, caused by excessive tumor growth in the absence of a sufficient vascular supply. The transcription factor HIF1-α [74] is rapidly induced following hypoxia and activates transcription of a plethora of stress response genes encoding angiogenesis-related growth factors such as VEGF, bFGF, PDGF, and TGF-β [75–77].
Algire was the first to observe vascularization in melanoma transplanted to the mouse in a transparent chamber in 1943 [78]. Preclinical models and clinical investigations have characterized primary melanomas and metastases as highly vascularized, and increased angiogenic capacity implies impaired patient prognosis [79–81]. The most prominent of the angiogenesis-related growth factors is vascular endothelial growth factor (VEGF) . As a response to stressful conditions such as hypoxia in a tumor, VEGF expression in melanoma cells is increased. Also, other cells in the tumor microenvironment, such as endothelial cells, CAFs, and inflammatory cells, express and secrete VEGF [82]. The total angiogenic response is dependent on many different growth factors (and inhibitors). In addition to VEGF, bFGF, PDGF, IL8, and PlGF act in concert with increased vascular supply as a result [82].
In 1983, vascular permeability factor (VPF) was purified [83] and later renamed as vascular endothelial growth factor A (VEGF-A) [84]. The VEGF-family consists of five members, VEGF-A to VEGF-D and placenta growth factor (PlGF), and belongs to the platelet-derived growth factor supergene family [85]. VEGF-A plays a key role in physiological and pathological angiogenesis, activates proliferation and migration of endothelial cells, and induces vascular leakage and vasodilatation [86]. Alternative splicing of the VEGF-A gene results in several pro-angiogenic isoforms (VEGF121, VEGF145, VEGF148, VEGF165, VEGF183, VEGF189, VEGF206) as well as anti-angiogenic VEGF-xxxb isoforms that have a different C` terminus [87]. VEGF165 is the most common isoform and is primarily heparin bound upon secretion [86]. Hypoxia, several cytokines, and growth factors as well as oncogenes and tumor suppressor genes regulate VEGF expression [86]. VEGF binds to the tyrosine kinase receptors VEGFR-1 and VEGFR-2 on endothelial cells and tumor cells; binding to VEGFR-2 is crucial in angiogenesis and activates further downstream signaling via phospholipase Cy and PI3K pathways [88]. Conversely, binding of VEGFxxxb to VEGFR-2 seems to inhibit the key functions of VEGF-A [87]. VEGF-A expression is described in primary melanomas as well as metastases [79].
A specific neovascular phenotype associated with VEGF has been identified in subgroups of melanoma, as well as other cancers, and is associated with aggressive tumor biology. Glomeruloid microvascular proliferations (GMPs) are focal aggregates of small vessels resembling a renal glomerulus. In mice, GMP has been induced by local injection of an adenovirus vector directing VEGF-A expression [89, 148]. A human parallel appears to be the POEMS syndrome [89], where increased VEGF-A is associated with glomeruloid hemangiomas. In humans, GMP is a defining histologic feature of glioblastoma multiforme and a prognostic factor in several other tumors [90, 91]. The presence of GMP’s in primary tumors has been associated with impaired prognosis [90].
Previously it was shown that inhibition of bFGF- and FGFR-1-mediated signaling abolished angiogenesis in human melanoma xenograft tumors [92]. In addition, bFGF expression was associated with higher microvessel density in primary melanomas [56]. There is evidence for a cross talk between FGF and VEGF signaling in angiogenesis [93].
Interestingly, not only endothelial cells have functional receptors for angiogenic factors. Melanoma cells have been observed to induce expression of growth factor receptors such as VEGFR as well as bFGF receptors [56, 79, 82]. This suggests the existence of autocrine and intracrine loops, in which melanoma cells produce VEGF in order to self-stimulate through its own VEGF receptors. VEGF secreted from melanoma cells also stimulates nearby endothelial cells in a paracrine fashion. Interestingly, in contrast to the paracrine stimulation by VEGF, the autocrine and intracrine stimulation was not inhibited by anti-VEGF-specific targeting by bevacizumab (an anti-VEGF antibody) [94]. This can, in part, explain why VEGF targeting drugs like bevacizumab can reduce microvessel density (MVD, quantification of angiogenesis in biopsies) yet still have only limited effects in clinical trials of melanoma [95–97].
As a rapid response to stress occurring in the microenvironment of melanomas, such as hypoxia, UV-light, as well as the omnipotent EMT inducer, TGF-β1, production of interleukin 8 (IL8) is induced. Increased level of IL8 in melanoma patients is associated with advanced disease and overall survival and is a potent stimulator of tumor angiogenesis, tumor progression, and metastasis [82, 98]. As mentioned above, the integrin αvβ3 is of particular significance in melanoma progression. VEGF- and bFGF-induced angiogenesis can be modulated by αvβ3 integrin in concert with its ligands vitronectin and osteopontin [99].
The pro-angiogenic role of heat-shock protein 27 (HSP27) was proven in cell-line studies, xenografts, and patient samples [100]. HSP27 belongs to the family of small heat-shock proteins that maintain cell survival under stressful conditions [101, 149]. Downregulation of HSP27 in an angiogenic breast cancer cell line resulted in decreased secretion of VEGF-A and bFGF and a non-angiogenic phenotype in vivo. Additionally, weak expression of HSP27 in human melanoma and breast cancer samples was associated with less aggressive phenotype and better outcome [100]. As many of the processes discussed in this chapter relate to normal cellular stress responses co-opted by melanoma cells, rapidly induced stress response proteins, like HSP27, might serve as promising treatment targets as well as biomarkers for prognosis and treatment response.
In 1999, Maniotis and colleagues presented a concept of vasculogenic mimicry (VM) in melanoma [101]. VM refers to the plasticity of aggressive cancer cells forming functional vascular networks, contributing to tumor perfusion by connecting to the normal endothelial-lined vasculature [102]. Although the very existence of VM as a functional fluid transporting network of channels has been heatedly debated and questioned [103], these studies have brought the field of angiogenesis forward and shed new light on the importance of tumor cell plasticity in aggressive tumors. VM has been validated as a prognostic marker in several tumor types, including melanoma [102], but can also be used as a marker of increased cellular plasticity and aggressiveness. Drugs to target cellular plasticity by inhibiting multiple signaling pathways simultaneously have shown some promise in preclinical studies. Combination treatment with anti-VEGF antibodies in conjunction with the Notch ligand anti-delta-like ligand 4 (Dll4) resulted in significant tumor growth inhibition in comparison to monotherapies [104], and anti Dll4 treatment might prevent resistance to anti-VEGF therapies [105]. Potentially, VM may serve as a relatively easily available marker of response to such treatment strategies.
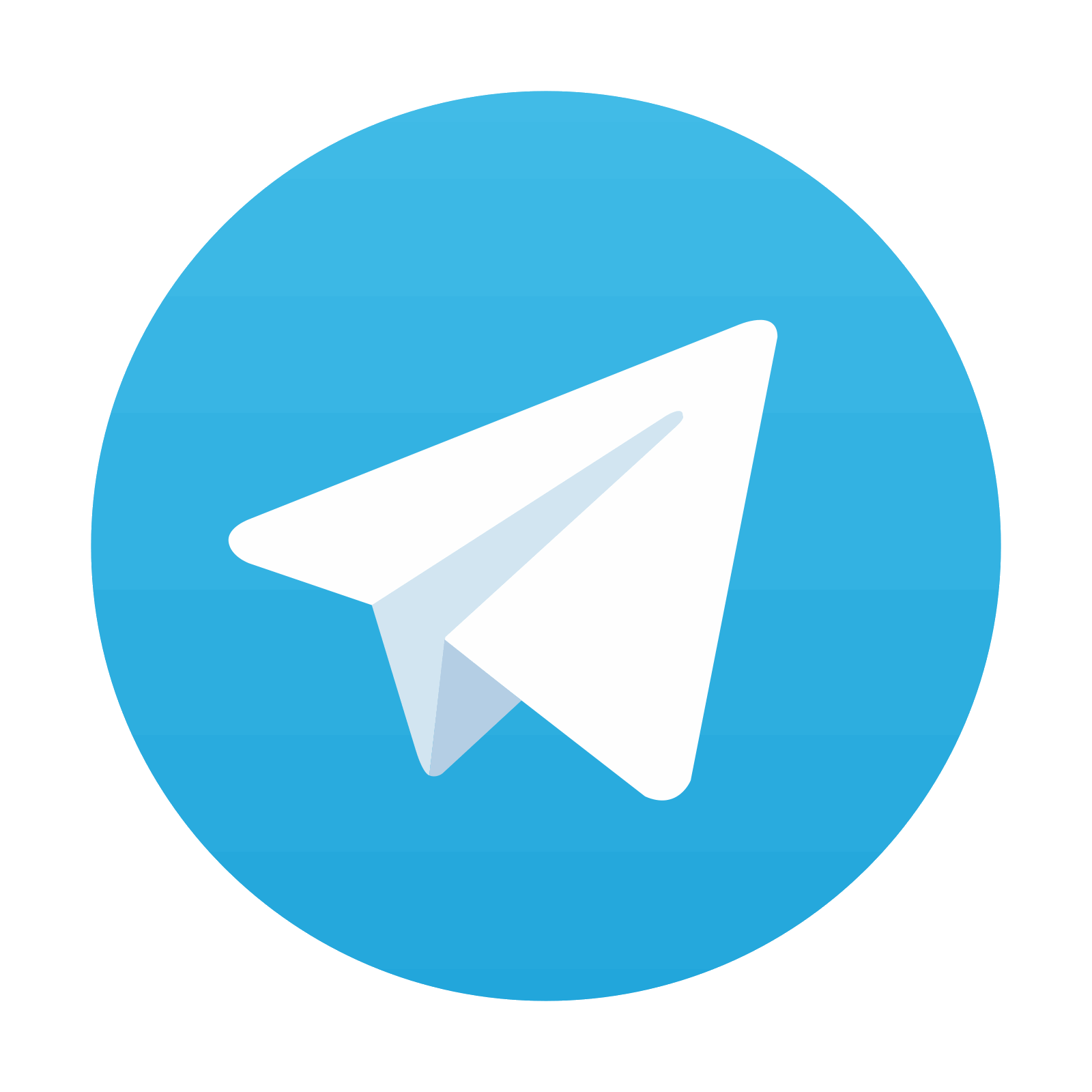
Stay updated, free articles. Join our Telegram channel
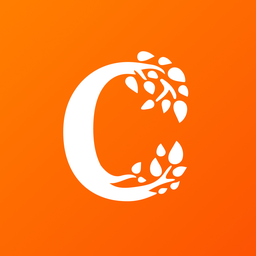
Full access? Get Clinical Tree
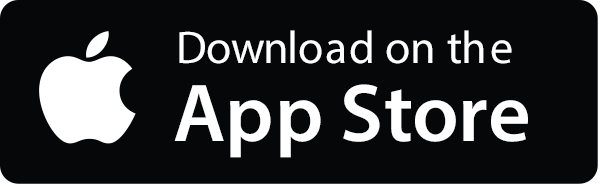
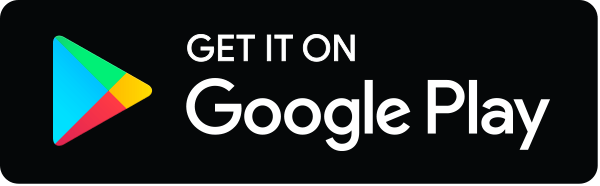