The Senses
The special sense organs of hearing, vision, taste, and smell, along with touch, play a vital role in protecting the human body. These organs also facilitate meaningful interaction with the environment. Each sense allows us to respond to subtle and not-so-subtle stimuli with precision and recognition. Because of the way our senses bring the external environment to us, the day-to-day implications of losing a sense are enormous.
●Physiologic Concepts
SIGHT
Stimulation of light-sensitive receptors in the eye, called photoreceptors, leads to the sense of sight. The response of the photoreceptors is transmitted to the brain by way of electrical signaling that passes through several levels of increasingly complex cell networks. Once the signals reach the brain, they are interpreted as a particular visual image based on the complexity of firing patterns, the rate of firing frequency, and the coding of color. Therefore, to have the sense of sight, one needs:
a functioning eye to receive a stimulus,
cells capable of coding the stimuli electrically,
intact neural pathways to transmit the electrical signal, and
a cerebral cortex capable of interpreting the signal as a meaningful image.
Structure of the Eye
The eye is composed of three layers including the sclera, the choroid, and the retina. A diagrammatic representation of the eye is presented in Figure 11-1. The outermost (anterior) portion of the eye consists of tough white connective tissue covering the eyeball, called the sclera. At the center of the eye, the sclera becomes a transparent membrane, the cornea. Light rays enter the eye through the cornea. By way of its natural curvature, the cornea bends the rays, causing the light to become less scattered and more focused on the underlying tissue. The image projected through the cornea is upside down and reversed right to left where it strikes the back of the eye. A venous sinus, the canal of Schlemm, lies at the junction of the sclera and cornea.
The choroid, a darkly pigmented and highly vascular membrane lying under the sclera, helps reduce light scatter. Directly under the cornea, the choroid becomes the iris. The iris is a colored membrane that gives the eye its tint. At the center of the iris is an area without pigment: the pupil. The cornea focuses light rays on the pupil. The diameter of the pupil is controlled by smooth muscles that innervate the iris. These muscles cause the pupil to constrict in the light and dilate in the dark. The muscles controlling the diameter of the pupil are innervated by parasympathetic and sympathetic nerves.
Parasympathetic stimulation causes the pupil to constrict, and sympathetic stimulation causes it to dilate. Varying pupil diameter determines the amount of light that passes deeper into the eye.
Parasympathetic stimulation causes the pupil to constrict, and sympathetic stimulation causes it to dilate. Varying pupil diameter determines the amount of light that passes deeper into the eye.
![]() FIGURE 11-1 Transverse section of the eyeball. (From Porth, C. (2005). Pathophysiology: Concepts of altered health states (7th ed.). Philadelphia, PA: Lippincott Williams & Wilkins.) |
Posterior to the iris and pupil is the lens. The lens is a curved transparent structure that further bends the light rays. By passage through the lens, light rays are focused exactly on the most posterior and sensitive portion of the eye, the retina. The shape of the lens is controlled by a muscle that allows the lens to focus both distant and close objects precisely on the retina. The retina contains the photoreceptors of the eye, the rods and cones that change light rays into the electrical messages that the brain interprets as vision. In the center of the retina is the macula, the site of the most acute and finely detailed vision. The retina also contains the cells that join together to form the optic nerve— the pathway by which the visual signal travels to the brain. The fovea centralis is a depression in the macula corresponding to the point of central vision. Between the lens and the retina, the eyeball is filled with blood vessels and a gelatinous fluid, called vitreous fluid.
Cells of the Retina
The structures of the eye anterior to the retina function primarily to focus the light rays scattered by a particular image exactly on the retina. Once the light rays strike the retina, the cells there have the job of changing the light signal into an electrical signal and then passing the signal to the brain. The cells of the retina that receive and transform the signal are the photoreceptors: the rods and the cones. The second class of cells in the pathway is the bipolar cells; bipolar cells receive the electrical signal from the rods and the cones and pass that signal to the third class of cells in the retina: the ganglion cells. The axons of the ganglion cells travel to the central nervous system as the optic nerve.
Rods and Cones
Rod-shaped photoreceptors are heavily concentrated on the periphery of the retina. Each rod is connected by way of a chemical synapse to a bipolar cell. The rods contain the photosensitive chemical rhodopsin, which is one of the four photopigments present in the retina (the other three are found in the cones). Rhodopsin decomposes when struck by light. When rhodopsin is decomposed, sodium permeability in the rod is reduced, leading to a hyperpolarization of the rod (i.e., the inside becomes more negative). Hyperpolarization decreases the firing rate of the rod on the bipolar cell. Normally, the rod inhibits the firing of the bipolar cell. When the rod is hyperpolarized by light, bipolar cell inhibition is removed, and the bipolar cell depolarizes. Depolarization of the bipolar cell causes an action potential to fire in the ganglion cell. Action potentials produced by the ganglion cells are sent to the brain via the optic nerve. Rods fire even with low levels of light and so function to provide night vision. They do not provide information regarding color.
Cone photoreceptors are heavily concentrated in the center of the eye. They are the only photoreceptors present in the fovea, an area located at the center of the macula. Each cone cell contains one of the other three photopigments, which, like rhodopsin, decompose when struck by light. As in a rod cell, decomposition of the photopigment in the cone cell hyperpolarizes the cone cell, removing its inhibitory influence from the bipolar cell and causing an action potential to fire in the bipolar cell. As before, the bipolar cell transmits this action potential to a ganglion cell that sends the signal to the brain via the optic nerve. Unlike rods, cones are insensitive to faint light and so contribute little to night vision. The photopigments present in the cone cells are color sensitive, allowing cone cells to transmit information regarding color, as described below. Vitamin A produces visual pigments and is an important component of both rhodopsin and the cone photopigments.
Reactivation of the Photopigments.
Immediately after decomposition, the original structure of the photopigments is returned. The rods and cones again inhibit the firing of the bipolar cells and are ready to respond to another light signal.
Differences Between Rods and Cones.
Rods are capable of responding to low levels of light; therefore, they provide limited vision in the dark. Many rods usually converge on one bipolar cell. The convergence of many rods on one bipolar cell reduces the acuity of rod vision, but increases its sensitivity. Rods are not color sensitive. Therefore, all stimulation is perceived in shades of gray. Cone stimulation requires higher levels of light and so cones do not fire in the dark or in near dark. Because few cones converge on any one bipolar cell, there is increased acuity of cone vision.
Color Vision.
The different photopigments of the cones allow for color vision because of their sensitivity to the colors red, blue, and green. The ratio of red, blue, and green cones activated at any one time results in color vision. Which cones are stimulated determines which bipolar cells depolarize and which ganglion cells fire action potentials. Ganglion cells may receive information from several different bipolar cells activated by one color-specific cone or a few different color-specific cones. Ganglion cells may be activated by one color, but inactivated by a second color. These variations and levels of stimuli allow for fine discrimination between many shades of color.
Lateral Neurons
Two types of neurons, the horizontal cells and the amacrine cells, lie laterally (sideways) in the retina and fire in such a way that they modify and control the messages being passed from the rods and cones to the bipolar cells and from the bipolar cells to the ganglion cells. Horizontal cells connect rods and cones to each other and to bipolar cells. Horizontal cells fine-tune the transmission of signals to the bipolar cells, thereby refining visual acuity. The
amacrine cells fine-tune signals between the ganglion cells, functioning to sharpen transient responses. Given the large number of lateral neurons in the retina, their importance in fine visual discrimination must be great indeed, although their exact mechanism of action is poorly understood.
amacrine cells fine-tune signals between the ganglion cells, functioning to sharpen transient responses. Given the large number of lateral neurons in the retina, their importance in fine visual discrimination must be great indeed, although their exact mechanism of action is poorly understood.
Optic Nerve
Ganglion cell axons join together to form the eye’s optic nerve (cranial nerve II). The optic nerve leaves the eye as a bundle through an area of the retina called the optic disk. The optic disk lacks rods or cones; therefore, it does not participate in the response to light (i.e., it is a blind spot). The central artery of the retina enters the eye through the optic disk. An area called the physiologic cup is at the center of the optic disk.
When the optic nerve reaches the brainstem, some fibers from the left eye cross and project to the right side of the brain. At the same time, some fibers from the right eye cross and project to the left side of the brain. This crossing at the optic chiasma allows both cerebral hemispheres access to information from each eye. Other fibers do not cross sides. The optic nerves terminate in the thalamus, in an area called the dorsal lateral geniculate nucleus, and there activate other neurons that then project to the occipital lobe. It is in the occipital lobe—the area of the brain that interprets the electrical signals as a meaningful visual image—that the visual cortex of the brain resides (Fig. 11-2). The integrity of the image from the dorsal lateral geniculate nucleus to the occipital lobe is ensured because each cell in the dorsal lateral geniculate nucleus passes the information in the same exact spatial arrangement to the visual cortex. The pathway of vision is shown in Figure 11-3.
Descending Inhibition to the Dorsal Lateral Geniculate Nucleus
Descending fibers from higher brain centers can influence the transmission of signals from the dorsal lateral geniculate nucleus to the visual cortex. These inhibitory and excitatory fibers come from the visual cortex itself and from areas of the brainstem. Descending stimulation can limit or accentuate what visual information is allowed to pass into consciousness.
Integration of the Visual Pathways
Integration of the pathways of vision occurs at each level of the retina: between the rods and the cones, at the horizontal cells, at the amacrine cells, at the bipolar cells, and at the ganglion cells. At each level, some cells fire with certain stimuli, such as specific on-off fields or various horizontal and vertical patterns, while the same stimuli turn off the firing of other cells. Ganglion cells have a complex pattern of firing that depends on the various on-off firing patterns of the bipolar cells and the amacrine cells. Bipolar cells are activated by unique combinations of the firings of rods, cones, and horizontal cells.
Continued integration of signals in the brain further refines the original, simple photoreceptor decomposition that initiated the cascade.
Optics of Vision
It is essential that the cornea and lens direct the light rays exactly toward the cells of the retina so that well-focused images can appear. Although the cornea must bend the rays initially, it is by changing the shape of the lens that the image is made to land exactly on the retina. This process is called accommodation. Accommodation occurs by the contraction and relaxation of the muscles that control the shape of the lens. A progressive loss in the ability of the lens to accommodate and focus the light rays occurs by approximately the fifth decade of life and is responsible for the loss of near vision in middle age (called presbyopia). Accommodation, pupil size, and eye movements are controlled by cranial nerves II, III, IV, and VI.
Intraocular Pressure
Pressure in the chamber of the eye is called intraocular pressure (IOP). IOP is tightly controlled at approximately 15 millimeters of mercury (mm Hg). The pressure is determined by the amount of a fluid, called aqueous humor, which fills the space between the lens and the retina. Aqueous humor is formed by a small gland, the ciliary body, located behind the iris. Once formed, aqueous humor flows through the eye, bathing all structures and providing nutrients to the lens and other cells. The aqueous humor then exits the eye through a venous channel between the cornea and the iris. This venous channel, the canal of Schlemm, joins extraocular veins that carry blood and fluid away from the eye.
External Structures of the Eye
The lacrimal glands (tear ducts), conjunctiva (mucous membrane layer on the outside of the eye), and eyelids make up the external structures of the eye. These structures protect the eye from irritants and injury. Tears also contain antimicrobial chemicals.
HEARING
The sense of hearing occurs when sound waves enter the external structure of the ear, pass through the middle ear to the inner ear, and stimulate specific receptor cells in the inner ear that fire action potentials, which in turn are carried to the brain. The action potentials are transmitted via the cochlear nerve (part of cranial nerve VIII) to the auditory cortex, a structure located in the temporal lobe of the brain (see Fig. 11-2), where they are interpreted as sounds. A diagrammatic representation of the ear is presented in Figure 11-4.
External Ear
The external ear consists of the auricle (outside cartilage) and the external ear canal. The auricle gathers the sound waves and projects them into the external canal. The external ear canal is a tube through which the sound waves travel to the middle ear. Separating the external ear from the middle ear is the tympanic membrane, also called the eardrum. A part of the temporal bone, the mastoid process, lies behind and below the external canal.
Middle Ear
The tympanic membrane is stretched tightly across the end of the external canal. When sound waves strike the eardrum, it is pushed in, or bowed, toward the middle ear. The degree of bowing of the eardrum depends on the loudness of the sound. After one sound wave, the eardrum returns to its previous position. The eardrum can be pushed in again and again if the sound waves
continue, causing the drum to vibrate. The frequency with which the eardrum vibrates depends on the frequency of the sound waves.
continue, causing the drum to vibrate. The frequency with which the eardrum vibrates depends on the frequency of the sound waves.
The middle ear has three bony processes, which are connected in series to the eardrum: the malleus (hammer), the incus (anvil), and the stapes (stirrup). Vibrations of the eardrum are transmitted from one small bone to the next, eventually striking the oval window. The oval window is a small membrane at the entrance to the inner ear. Because the oval window is smaller than the tympanic membrane, the force of the sound waves on the oval window per unit of area is significantly magnified.
The middle ear is connected to the nose and throat via the eustachian tube. Although normally closed, the eustachian tube opens with yawning or swallowing. This opening allows the pressure in the middle ear to remain equal to atmospheric pressure.
Inner Ear
The inner ear is a complex organ consisting of two mazelike structures: the outer bony labyrinth and the inner membranous labyrinth. The bony labyrinth is separated from the membranous labyrinth by a thick fluid called the perilymph. The membranous labyrinth is filled with a slightly different
fluid called endolymph. The bony labyrinth contains the cochlea, the vestibule, and the semicircular canals. The cochlea is the organ responsible for changing sound waves to action potentials. The vestibule and the semicircular canals maintain equilibrium and balance, which is a major function of the inner ear.
fluid called endolymph. The bony labyrinth contains the cochlea, the vestibule, and the semicircular canals. The cochlea is the organ responsible for changing sound waves to action potentials. The vestibule and the semicircular canals maintain equilibrium and balance, which is a major function of the inner ear.
Cochlea
The cochlea is a shell-shaped organ, filled with perilymph. The cochlea is separated down the middle by a structure called the basilar membrane. On the basilar membrane is a blanket of hair cells that, together with the basilar membrane, compose the organ of Corti, which is the organ of hearing. The hair cells on the basilar membrane depolarize when deformed or bent. Each hair cell synapses upon an afferent neuron, the axons of which make up the acoustic nerve. Depolarization of a hair cell initiates a receptor potential, which, if large enough, stimulates an action potential in the afferent neuron. The hair cells are covered by an overhanging membrane, called the tectorial membrane. It is against the tectorial membrane that the hair cells bend when a sound wave is passed into the inner ear.
Sound Wave Transmission
When a sound wave strikes the oval window, a pressure wave is generated in the fluid-filled inner ear. The pressure wave causes a wavelike displacement of the basilar membrane against the overhanging tectorial membrane. As the hair cells rub against the tectorial membrane, they are bent. This bending leads to the depolarization of the hair cell and the production of a receptor potential. With significant deformation, the afferent nerves synapsed upon by the hair cells are stimulated to fire action potentials and the signal is transmitted to the auditory cortex. The pathway of hearing is shown in Figure 11-5.
The frequency of the pressure wave determines which hair cells are displaced and, consequently, which afferent neurons fire action potentials. For instance, the hair cells lying on the part of the basilar membrane nearest to the oval window are most displaced by high-frequency sounds, whereas the hair cells lying on the basilar membrane farthest from the oval window are most displaced by low-frequency sounds. The brain interprets the pitch of a sound by which neurons are activated. The brain interprets the intensity of a sound by the frequency of neuronal impulses and the number of afferent neurons firing.
Vestibular System and Equilibrium
The vestibule and the semicircular canals also contain hair cell receptors that are sensitive to movement and position. The crista ampullaris is located in the canals and each time the head is moved, nerve impulses are generated. When the head is turned, the hair cells are bent as they pass through the thick endolymph surrounding them. As in the organ of Corti, bending of a hair cell in the vestibule and semicircular canals causes depolarization of the cell and the firing of an action potential. Action potentials initiated in the vestibule and semicircular canals are carried via the vestibular nerve to the parietal lobe of the brain, converging
near the somatosensory area, where information on joint and muscle position is integrated (see Fig. 11-2). The semicircular canals and the vestibular apparatus work together with other tactile and visual systems to determine the current position of the body and any change in motion or direction.
near the somatosensory area, where information on joint and muscle position is integrated (see Fig. 11-2). The semicircular canals and the vestibular apparatus work together with other tactile and visual systems to determine the current position of the body and any change in motion or direction.
TASTE
Chemical receptors that generate impulses resulting in taste are called taste buds. Taste buds are located in a pattern on the tongue and are depolarized in response to specific chemical stimulation. Gustatory cells in the taste buds are the sensory receptor cells responsible for generating nerve impulses. Depolarization of the taste buds leads to action potentials and the firing of cranial nerves V, VII, IX, and X. These nerves send their information to the taste cortex in the parietal lobe (Fig. 11-2), where the sensation is identified. The pathway of taste is shown in Figure 11-6.
There are specific taste buds for many different taste sensations, some of which are as yet unidentified. Known taste receptors are usually divided into those which respond to sweet, bitter, salty, and sour tastes. Research is suggesting that the metallic taste and a “meaty” or “savory” flavor activated by amino acid glutamate may also be considered primary tastes. Activation of different receptors to varying degrees by substances found in food allows for a wide range of tastes. The sense of taste initiates digestion and provides a stimulus to eat. There can be adaptation (decreased firing) of the taste buds if exposure to a chemical stimulus is prolonged. Certain drugs, including nicotine, may sensitize some receptors while desensitizing others, causing taste sensations to change. Another important component influencing the sense of taste is smell.
OLFACTION
The sense of smell is provided by receptor cells, called olfactory cells, which line the membranes of the nasal mucosa. The olfactory cells contain cilia
that depolarize when bound by certain chemicals corresponding to specific odors in the air. A few types of cilia hyperpolarize in response to any one specific odor. Significant depolarization or hyperpolarization of the cilia leads to the firing of action potentials in the neurons of the olfactory nerve (cranial nerve I) that terminate in the olfactory bulbs of the frontal lobe. From there, the signal is passed to the olfactory cortex in the limbic system of the brain (see Fig. 11-2). The olfactory receptor cells adapt rapidly to a continuing smell. The pathway of smell is shown in Figure 11-7. Progressive reduction in the sense of smell can occur from the pollutants of tobacco smoke as well as the aging process.
that depolarize when bound by certain chemicals corresponding to specific odors in the air. A few types of cilia hyperpolarize in response to any one specific odor. Significant depolarization or hyperpolarization of the cilia leads to the firing of action potentials in the neurons of the olfactory nerve (cranial nerve I) that terminate in the olfactory bulbs of the frontal lobe. From there, the signal is passed to the olfactory cortex in the limbic system of the brain (see Fig. 11-2). The olfactory receptor cells adapt rapidly to a continuing smell. The pathway of smell is shown in Figure 11-7. Progressive reduction in the sense of smell can occur from the pollutants of tobacco smoke as well as the aging process.
TOUCH
Tactile sensations include the body’s recognition of touch, pressure, and vibration. Each of these sensations appears to be mediated by receptors that vary only in location; touch receptors are located in or near the skin, whereas pressure receptors are found deeper in the tissues. Vibration is sensed as rapidly repeating stimuli activating both touch and pressure receptors.
Tactile Receptors
There are several types of tactile receptors spread over the body. Tactile receptors are mechanoreceptors, cells that respond to physical deformation and compression with depolarization, causing a receptor potential. If the depolarization is great enough, the nerve fiber attached to the receptor fires an action potential and transmits the information to the spinal cord and the brain. Different tactile receptors vary in sensitivity and in the velocity with which they send their impulses. They also vary as to which type of nerve fiber transmits their signal to the spinal cord and the brain.
Peripheral Nerve Fibers that Transmit Tactile Information
Tactile sensation is carried to the spinal cord by one of three types of sensory neurons: large type A beta (β) fibers, smaller type A delta (δ) fibers, and small type C fibers. Both types of A fibers are myelinated, transmitting action potentials rapidly; the larger fibers transmit faster than the smaller fibers. Tactile information carried in the A fibers is typically well-localized and pinpoint. The small C fibers are unmyelinated, transmitting action potentials to the spinal cord much more slowly than the A fibers. Tactile information carried in the C fibers is poorly localized.
Transmission of Tactile Information in the Spinal Cord
Virtually all information on touch, pressure, and vibration enters the spinal cord via the dorsal roots of the corresponding spinal nerve. After synapsing in the spine, highly localized information carried in the fast-firing A fibers (both β and δ) is sent to the brain by way of the dorsal column-lemniscal system. Nerve fibers in this system cross over left to right in the brainstem and travel through the thalamus before synapsing in the somatosensory cortex (see Fig. 11-2). Information on temperature and poorly localized touch is carried to the spinal cord by way of the slow-firing C fibers. This information is sent to the reticular area of the brainstem and then to higher centers via fibers carried in the anterolateral system. Pain and some sexual sensations are transmitted in the anterolateral tracts.
Types of Tactile Receptors
There are six basic types of tactile receptors: free nerve endings, Meissner corpuscles, expanded-tip tactile receptors, hair end-organ receptors, Ruffini
end-organ receptors, and Pacinian corpuscles. These six types of tactile receptors are discussed individually in the following sections.
end-organ receptors, and Pacinian corpuscles. These six types of tactile receptors are discussed individually in the following sections.
Free Nerve Endings
Receptors that respond to touch are found all over the skin and are called free nerve endings. Most send their information to the spinal cord via the small type A δ fibers. From the spinal cord, information from the free nerve endings is sent through the thalamus to the somatosensory area (parietal lobe) of the cortex. Some free nerve endings send their information to the cord via the slow type C fibers. Free nerve endings respond to stimuli perceived as painful.
Meissner Corpuscles
Touch receptors found on areas of the body not covered with hair, especially the fingertips and lips, are called Meissner corpuscles. These receptors allow for precise discrimination concerning the location of a touch. Information from Meissner corpuscles is carried to the spinal cord via fast-firing type A β nerve fibers. From the spinal cord, information from Meissner corpuscles is sent through the thalamus to the somatosensory area of the cortex.
Expanded-Tip Tactile Receptors
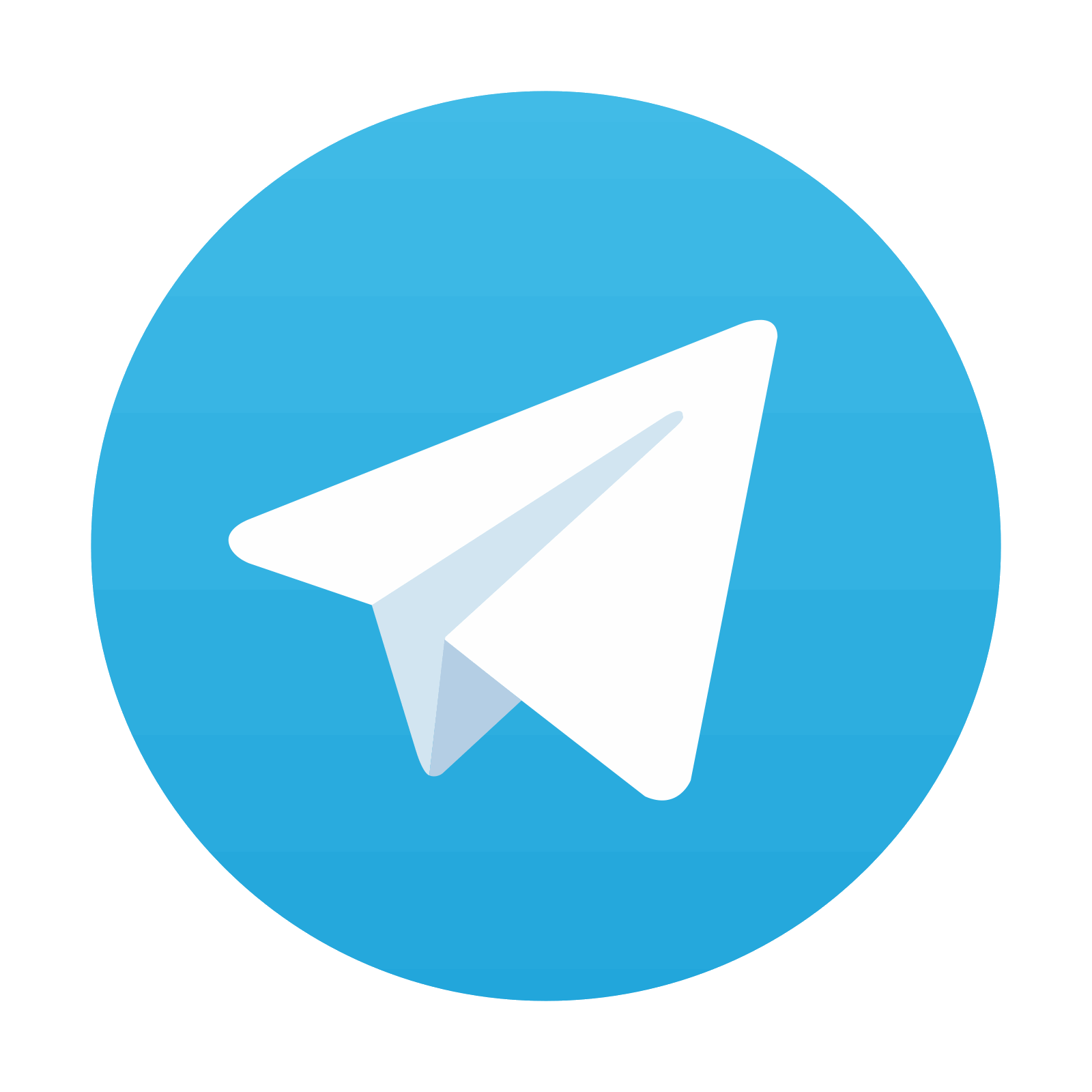
Stay updated, free articles. Join our Telegram channel
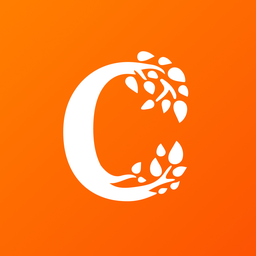
Full access? Get Clinical Tree
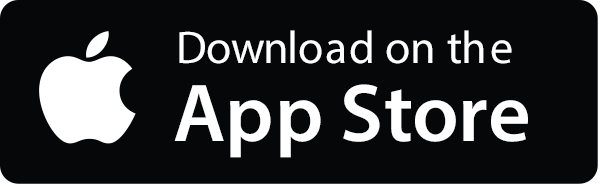
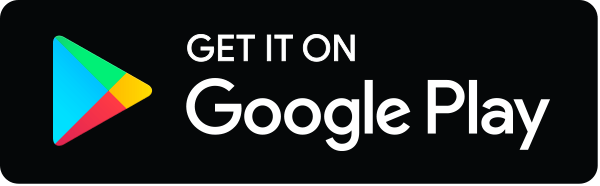
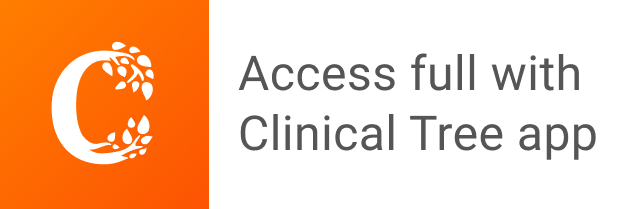