The Brain
If the human brain is compared to the brains of other species, there are two major differences that will be noted. First of all, the size of the human brain is relatively large. This statement is perhaps obvious if one were to compare a mouse brain to a human brain; but even if brain size is considered in relation to body size, the human brain is still comparatively large. In addition to differences in size, the human brain differs from the mouse brain in shape. In particular, the cerebrum of the human brain is much larger (Figure 4.1.). This enlargement is especially evident in the front lobes of the brain. This difference in human brain size and structure is the physical basis of the many behaviours – for example, language – which differentiate humans from other species. The first stage in our consideration of the physical basis to communication is to explore the human brain. We can do this on both a macro- and micro-level. At a macro-level we can see how the brain can be divided into a number of component structures – for example, its hemispheres and lobes. We can then go on to look at its micro-structure, investigating the brain at the level of the cell. Just as the brain can be divided into different structures, so too can brain cells, which belong to different families, and vary greatly in structure. We shall begin our consideration of the brain at the level of its macro-structure.
Figure 4.1. The brain and spinal cord
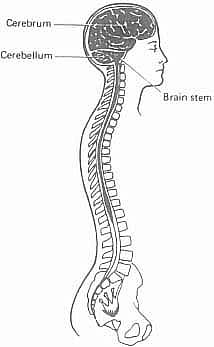
The network of connections whereby signals can be transmitted from one part of the body to another is known as the nervous system. This system is subdivided into the central nervous system (CNS) and the peripheral nervous system (PNS).
Central nervous system
The CNS consists of the brain, the spinal cord which connects to it, and their associated membranes and fluids. The system is immensely complex, and its potential for processing information needs to be appreciated. It contains billions of nerve cells, some of which relay information to and from connections with the peripheral nervous system, others of which transfer information within the central nervous system. The brain is contained within the skull, or cranium, whilst the spinal cord is the elongated tube which runs for some 45 cm within the vertebrae of the spine. The relationship of the brain to the spinal cord is illustrated in Figure 4.1.
Peripheral nervous system
As its name suggests, this system of nerves links the CNS with the rest of the body. Sensory information is relayed from a variety of receptors (e.g. touch, taste, kinaesthetics, pain) to the junction with the CNS. In addition, instructions from the central system are transferred to nerves for transmission to the appropriate effectors, e.g. a muscle. Peripheral nerves which leave the CNS from the spinal cord are known as spinal nerves. There are 31 pairs of these nerves – each pair leaving the spinal cord at the level of each vertebra. A second set of peripheral nerves leave the CNS from within the cranium and are attached directly to the brain. These are called cranial nerves. There are 12 paired cranial nerves, and they are essential for the control of speech, and for relaying information from the ear back to the brain.
We now need to proceed to a more detailed study of the brain, because in Chapter 5 we shall be referring to several categories of disability that are the result of damage to specific brain areas. But it will be useful, first, to introduce a terminological preliminary. In order to describe any area of human anatomy, it is necessary to use a terminology about which there is universal agreement, otherwise there would be ambiguity in knowing which specific part of the brain, for example, was being referred to. What would ‘on the left side’ mean, for instance? ‘Left’, from the point of view of the observer, or of the person whose brain it is? To avoid such problems, it is conventional to see the human body as capable of being divided along various lines, or planes. Thus, we can imagine a line vertically dividing the front part of the body from the back part, as in Figure 4.2a: this is known as the coronal or frontal plane; and it divides the body into anterior or ventral (i.e. front) and posterior or dorsal (i.e. back) parts. Second, we can imagine the body being divided vertically into left and right sides, as in Figure 4.2b: this is known as the sagittal plane; and if the division has been made exactly in the middle (in the midline or median), it is referred to as the medial sagittal plane. Lateral refers to a plane further away from the midline; medial to a plane closer to the midline. The terms ‘left’ and ‘right’ are always used from the point of view of the body being described, and not as from the observer. Third, we can imagine a line dividing the body horizontally, at right angles to its vertical axis, as in Figure 4.2c: this is referred to as a transverse or horizontal plane. Fourth, relative position on the vertical axis is referred to using the terms superior (for higher up) and inferior (for lower down). Fifth, a view of the body from underneath, looking upwards, is referred to as a basal view: see Figure 4.2d. Lastly, a feature further away from a point of origin is said to be distal; one nearer to the point is proximal.
Figure 4.2. Anatomical planes: (a) coronal; (b) sagittal; (c) transverse; (d) basal
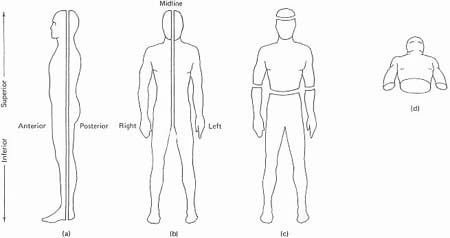
Using this terminology, we can now begin to identify those areas of the brain that are of particular importance for the study of linguistic disability. A general point to be made, first of all, is that the brain is not a single, undifferentiated structure, but contains several anatomically distinct regions. The largest part of the brain is known as the cerebrum (Figure 4.1.). Its most noticeable feature is the way it is divided sagittally into two great lobes of similar size, the cerebral hemispheres. There is, accordingly, a left hemisphere and a right hemisphere, the differential function of which we shall discuss below. Unilateral refers to one of these hemispheres alone, bilateral to both hemispheres; contralateral to the opposite side of the body from a particular hemisphere. Beneath the hemispheres is a thumb-sized midline structure, the brain stem, which connects the two hemispheres to the spinal cord. It is described as having three parts: the midbrain (from which the hemispheres spring), the pons and the medulla oblongata, which continues below as the spinal cord. Arising dorsally from the pons is another major structure, the cerebellum, which is responsible for the maintenance of body posture and the smooth co-ordination of all movements, including walking and speaking. Buried deep within the hemispheres are further masses of nerve tissue, the basal ganglia (which help to control movement), the limbic system (which consists of structures such as the hippocampus and amygdala, important for memory) and the thalamus (which relays information to and from the cortex, and helps to analyse sensory information). The relationship between the hemispheres is shown from above in Figure 4.3a, laterally in Figure 4.3b, and that between the other structures is shown in medial sagittal section in Figure 4.3c.
Most research relevant to language processing has focused on the structure and function of the cerebrum, and in particular on its surface layer of grey matter (grey, because the cell bodies of the cerebral neurons are concentrated here) known as the cerebral cortex.2 The reason for this emphasis is that the cortex is the part of the brain which seems to be primarily involved in the decoding of information from the senses and in the control of voluntary movement and intellectual functions. It is also the area of the brain most amenable to direct observation and investigation. It contains about 10 billion cells and some 200 million fibre processes. Beneath the cortex is a body of white matter, which transmits signals between cortex and brain stem, and from one area of the cortex to another. The most noticeable feature of the cortex is its lack of a smooth surface: what has happened is that, during its course of development (from between the third and fourth months of life in the womb) the surface of the brain has folded in upon itself along certain genetically predetermined lines. A large area of cortical tissue is thus packed into a relatively small anatomical space. The various folds that have been produced are known as convolutions or gyri (singular: gyrus). The furrows on either side of the gyri are known as sulci (singular: sulcus). Each has a precise anatomical designation, but for present purposes we need concern ourselves only with the most dominant features, and those which are most relevant for language.
Figure 4.3. (a) The brain from above; (b) right lateral view; (c) relationship between the main brain structures
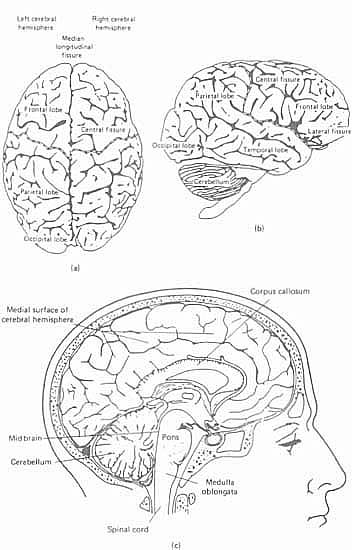
The brain is most commonly represented diagrammatically from above and laterally. The former view has been given in Figure 4.3a; a lateral view (left lateral) is given in Figure 4.4. The dominant feature of the former view is the deep fissure, known as the median longitudinal fissure, which separates the hemispheres. It does not, however, extend the whole way down through the cerebrum; the two hemispheres are in fact joined deep within the cerebrum by a thick bundle of nerve fibres, known as the corpus callosum (see Figure 4.3c) the means whereby information from one hemisphere can be transmitted to the other. The dominant features of the lateral view are also fissures, or sulci:3 the central sulcus, fissure of Rolando, or Rolandic fissure (named after the Italian anatomist, Luigi Rolando (1733-1831)), and the lateral sulcus, or Sylvian fissure (after the Flemish anatomist, François de la Boë Sylvius (1614-1672)). These fissures are of particular importance, because they are used as the primary anatomical basis for dividing the brain up into different major areas or lobes. Four such lobes are universally recognized, within each hemisphere, based on the topographical relation of these areas to the skull (the temporal lobe corresponds to the temporal bone, etc.):
Figure 4.4. Superolateral surface of cerebral hemisphere
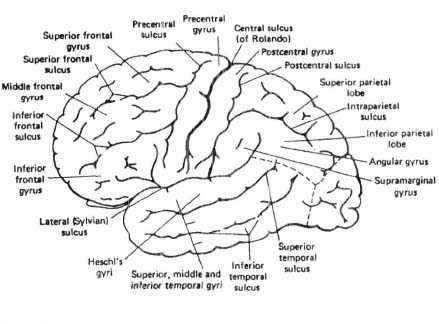


These are all indicated in Figure 4.4.
Up to this point, our discussion of the CNS has been in terms of macro-structure. But we can also examine the component parts of the nervous system and study their internal structure. A nerve cell is known as a neuron or neurone (both spellings are in current usage). The actual structure of the cell varies across regions of the CNS – for example, distinctive cells can be found within the thalamus or basal ganglia. But all cells share certain common characteristics; and their function is to conduct electrical impulses. The structure of a neuron is illustrated in Figure 4.5. Each neuron has two main constituents: a cell body (sometimes called the soma or cyton), which contains the nucleus of the cell; and one or more branching processes4 which conduct impulses to and from the cell body. These processes are generally referred to as nerve fibres; they vary in length from a few thousandths of a millimetre (or micrometre) to over a metre, the longest connecting the spinal cord to the extremities of the hands and feet. One process conducts impulses away from the cell body; this is known as the axon, which is readily identified by its relative lack of branches along most of its length. The other processes conduct impulses into the cell body: these are usually several in number, much shorter than the axon, and containing several branches; these processes are known as dendrites. The type of neuron illustrated in Figure 4.5 is one that constitutes the majority in the nervous system (it is known as a ‘multipolar’ neuron).
Figure 4.5. Structure of a neuron
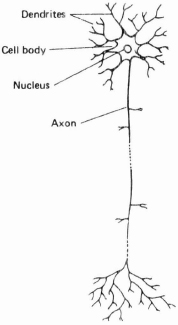
The direction in which impulses flow provides the basis for a binary classification of neurons into motor and sensory types. Motor neurons (or motor nerves) carry signals away from the CNS (i.e. they are ‘efferent’ nerves),5 either to muscles which they cause to contract, or to glands which they cause to secrete. Muscles and glands are effector organs – they produce the effective end result of nerve impulses. Sensory neurons (or sensory nerves), by contrast, carry signals from receptor nerve endings in the skin and other tissues, which respond to different stimuli (pain, touch, temperature, etc.) into the centre (i.e. they are ‘afferent’ nerves). One sequence of neurons transmits a signal from receptors to the brain; another sequence transmits signals in the reverse direction. This binary classification, however, is not exhaustive: another group of neurons exist that have relatively short axons and are responsible for sending signals within the CNS. Neurons connect at junctions known as synapses (singular: synapse /’sainæps/): a neuron is said to synapse with another neuron, or with a receptor cell, or an effector cell (such as a muscle fibre). The electrical impulse carried by the neuron triggers the release of transmitter substances at the synapse (these substances are known as neurotransmitters). These travel across the junction and influence the second neuron. The effect may be excitatory, encouraging the firing of the second neuron, or inhibitory, decreasing the likelihood that the second neuron will fire. The second neuron receives a very large number of synaptic inputs, some of which may be excitatory and others inhibitory. Thus neuron relays are not simple systems: whether the second neuron fires is dependent on a complex interplay of excitatory and inhibitory impulses.
If a neuron does fire, the electrochemical impulses last for about a thousandth of a second. The speed at which they travel along the neuron is very variable – some impulses travel at less than a metre per second; others are much faster, reaching speeds of around 100 metres per second. The factors that govern the rate of impulse transmission are several – the type of fibre involved, its general environment, and above all the nature of its protective covering. Most fibres are covered in a white, fatty substance called myelin; the covering as a whole is referred to as a ‘myelin sheath’. The sheath is about one millionth of a centimetre thick, and acts as an insulator for the fibre. However, this surface covering is not total: every half millimetre or so the covering is broken, at points known as ‘nodes of Ranvier’ (after the French pathologist, Louis-Antoine Ranvier (1835-1922)), and there are also variations in the thickness of the sheath along different fibres. The significance of this is that the presence of myelin affects the rate at which impulses travel: the greater the distance between the nodes, the faster the rate of transmission; also, the thicker the fibres, the faster the rate of transmission. Moreover, nerves develop their myelin sheaths (or become ‘myelinated’) at different periods in human development. All fibres are ‘unmyelinated’ to begin with; the process of myelination continues into adolescence, and proves to be an important factor in the extent to which the nervous system is capable of transmitting efficiently several of the more complex voluntary movements. For example, it is possible that the relatively late appearance of certain types of sound in speech (e.g. with high acoustic frequency, such as [s]) may be due to the degree of myelination of the auditory nerve and the cortical areas to which it connects (see further below), controlling the ability of the child to discriminate these sounds auditorily.6 It should also be noted that there are a range of diseases which specifically affect myelin, the most well-known of these ‘demyelinating diseases’ being disseminated (multiple) sclerosis.
Language and the Brain
At this point, we will try to relate what we have said about a particular human behaviour – language – to what is known about the structure and function of the brain. There has been an enormous amount of research in this area, but despite this there is much which is still unknown or speculative in our understanding of language-brain correlations. What is quite clear is that many production and reception stages of the communication chain are mediated by the brain. Messages are planned and linguistically encoded in the cortex; a number of regions of the brain are involved in motor programming and execution; and similarly, much of message decoding, from perception to interpretation, is mediated by the brain. It follows, therefore, that damage to the brain has the potential to cause failures both in message encoding and decoding.
Lateralization of functions
The most notable feature of the cerebrum, referred to above, is the way in which it is split into two hemispheres, of roughly equal size and proportions (there are certain asymmetries between the hemispheres, but these need not concern us here). The functions of the two hemispheres are not identical. Certain functions – for example, movement and sensation – are largely crossed functions: that is the left-hand side of the cerebrum controls the right-hand side of the body, and vice versa. Similarly, with sensory abilities – such as touch or vision – information from the right side travels, very largely, to the left hemisphere for processing. In addition to controlling and receiving information from different halves of the body, the cerebral hemispheres differ in other ways. In the past, the left hemisphere has been described as the dominant hemisphere, and the right relegated to a more minor role as the non-dominant hemisphere. This view was influenced by the fact that most people are right-handed -thus, it is argued, a right-hand, and so left-hemisphere, preference in handedness reflected a left-hemisphere dominance in all functions. Similarly, damage (or lesion) to the left hemisphere was observed to have catastrophic effects on that most public of functions – language; whilst lesion of the right hemisphere led to more subtle deficits which can often be revealed only by careful and sophisticated assessment. There have been changes in this view over the years, and it is now recognized that each hemisphere has specific processing strengths or patterns of dominance.7 The left hemisphere is important for language processing – an observation first made by Pierre Broca (see p. 107) in 1865, who noticed that brain lesions which caused language difficulties followed damage to the left hemisphere. Individuals who suffer right-hemisphere lesions do not experience obvious language deficits. The right hemisphere is important in processing visual and spatial information, such as knowing where we are, or using a map, and other complex visual abilities such as recognizing faces. It also plays a role in processing emotional information.
Evidence for this lateralization of functions comes from a variety of sources. The earliest methods were lesion studies – such as Broca’s – where patients who had suffered strokes or head injuries were the source of data. The behavioural deficits of patients with unilateral brain lesions (i.e. to the left or right hemisphere) were investigated, and behaviours which were absent or impaired were viewed as being controlled by the damaged hemisphere. Thus, as language deficits were identified following left-hemisphere damage, language was seen as a left-hemisphere function. Conversely, damage to the right hemisphere was typically associated with complex spatial disturbances – individuals had difficulty in copying designs or drawing, showed problems in navigation even within familiar environments, and had impairments of recognizing familiar faces.
This model of the functions of the two cerebral hemispheres is, however, not yet adequate. There is a further factor which needs to be considered – that of handedness, or laterality. This model of the left hemisphere as the language-mediating hemisphere and the right as a non-linguistic hemisphere holds for the vast majority of right-handed people (98%). But the situation in left-handed people is less clear. A significant proportion of left-handers are also left-hemisphere dominant for language (about 60%). The remaining 40% of left-handers are either right-hemisphere dominant for language and so can suffer from a language impairment following a right-hemisphere lesion, or appear to have bilateral language representation and experience linguistic difficulties following either a left- or right-hemisphere lesion. The relation between language and hemispheric organization is therefore complicated. The situation becomes yet more complex in the light of recent research which shows that damage to the right hemisphere, even in right-handers, can result in subtle impairments in communicative abilities. The type of behavioural deficits that have been identified in these patients include conversational disabilities – the patient’s conversation is described as rambling and irrelevant. Abnormalities in prosody, facial expression and eye contact have been recorded. Subtle aspects of language understanding such as interpreting humour and metaphor are impaired.8 It is not yet clear, however, whether the communicative difficulties which follow damage to the non-language dominant hemisphere are impairments specific to language, or whether they result from disruption of the cognitive functions such as attention and planning which are necessary to support the normal use of language.
In addition to the study of the effects of naturally occurring lesions, there are other sources of evidence regarding hemispheric specialization. One results from the surgical removal of a diseased hemisphere (hemispherectomy), or temporarily anaesthetizing one hemisphere (for instance, by injecting sodium amytal into the artery supplying the hemisphere); another results from the study of severely epileptic patients who have undergone surgery to cut the corpus callosum, the fibres connecting the two hemispheres (commisurotomy), in so-called ‘split-brain’ studies. In these instances it is possible to present stimuli to a single hemisphere, either because its fellow is anaesthetized, absent, or disconnected, and see how efficiently the isolated hemisphere can process information of a certain type – for example, linguistic or spatial information. The problem with all these procedures is that the patients who are subjected to them have a history of early brain damage. The young brain has a degree of plasticity of function; hence, if an important function is disrupted by a lesion early in life, other brain areas will take up the lost function. This means that the patterns of brain organization in individuals who undergo the major surgical procedures of commisurotomy and hemispherectomy are likely not to be typical of those of normal or undamaged brains. The validity of the assumption that the findings of studies with such subjects can be generalized to the normal brain is therefore questionable.
Other techniques have been developed to investigate processing in the normal, undamaged brain. The electroencephalograph (EEG) measures the electrical activity of the brain. Different types of stimuli can be presented to subjects and subsequent electrical activity of the brain monitored by sensors placed on the scalp. Activity can be compared between hemispheres or within a hemisphere. More evidence is drawn from experiments which exploit the crossed organization of the two hemispheres – the transmission of sensory information from the right side of the body to the opposite (or contralateral) hemisphere, and vice versa. Auditory, visual or tactile stimuli can be presented to one side of the body on the assumption that it is most rapidly processed by the opposite hemisphere. In a dichotic listening task, for instance, competing auditory stimuli are played simultaneously into each ear. Subjects are required to report what they have heard. The accuracy of their report varies with the nature of the stimuli. If the stimuli are words, then those played to the right ear (and hence the left hemisphere) are better reported whilst if the stimuli are complex tonal or musical information, these are more accurately reported via the left ear (and hence the right hemisphere).
An exciting new development in studying the areas of the brain responsible for certain types of processing is new techniques of brain scanning or brain imaging. It has been possible since the 1970s to produce images of living brain structures through the use of CT (computerized tomography) and, more recently, MRI scans (magnetic resonance imaging). A new generation of brain imaging techniques permits the recording of brain function. Functional MRI scanning and PET (positron emission tomography) scanning measure the activity of different regions of the brain. PET works by radioactively labelling substances, such as glucose, which are necessary for cell metabolism. The labelled substances are then administered to the patient and their uptake by regions of the brain is recorded. Areas which are active in processing have a greater demand for the radioactively labelled substance than those which are not. Functional MRI is a technique which does not involve exposing a subject to radioactive material. The technique rests on the fact that oxygenated blood and deoxygenated blood respond differently in strong electromagnetic fields. It is possible, therefore, to detect areas of the brain which have high concentrations of deoxygenated blood, which in turn suggests that the brain cells in that region have been particularly active in processing information. Both techniques allow brain activity to be measured, and the patterns of brain metabolism can be compared across activities – for example, differences between a resting state and a time when the patient is listening to words. Where an increase in activation occurs, it would be hypothesized that these regions are involved in the processing of a particular type of information. Functional brain scans show that, during language processing, large areas of the left hemisphere around the lateral sulcus are active, and that there is also activation in the parallel areas of the right hemisphere.
The methodological and theoretical problems of doing research in this area are very great. All the techniques that we have discussed have limitations attached to them. Ideas about lateralization of functions in the cerebral hemispheres have undergone much change. From viewing the right hemisphere as ‘non-dominant’, researchers recognized that the right hemisphere is specialized in processing certain types of information, while the left specializes in others. In particular, language was regarded as a left-hemisphere function. Contemporary investigations are now suggesting that the role of the right hemisphere in communication has to be re-evaluated in the light of evidence of conversational disability and subtle language impairments in patients with right-hemisphere lesions. In all of the above, there is a complex interaction between hemispheric specialization and handedness – that is, left- and right-handers may differ in their patterns of cerebral dominance.
Localization of functions
We have seen that the hemispheres of the cerebrum do not have duplicate functions. The next stage is to ask whether, within a single hemisphere, regions have identifiably different functions – that is, can functions be ‘localized’ to different parts of the hemisphere? The answer to this question seems to depend on the type of function that is under consideration. In our discussion of neuropsychology (p. 56) we have already touched upon the controversy over the extent to which cognitive functions can be precisely localized within a hemisphere. It would seem that some aspects of lower-level functions, such as movement and sensation, are capable of quite precise localization. For example, the area of the cortex which governs movements of a particular group of muscles can be identified with some precision (p. 108). But even with functions such as movement, a number of brain areas are involved in the planning and implementation of actions. Models of movement control usually include areas of the frontal lobe near to the central sulcus, the cerebellum, and basal ganglia, together with the various neurons that run between these areas and on down to the muscles. The brain areas involved in controlling higher cognitive functions such as language, memory, and thinking are even more complex. The discussion of models of language in Chapter 2 suggested that language can be subdivided into components such as grammar, semantics, phonological form and pragmatics. These different components may be underpinned by different brain systems, often in widely distributed locations. Thus it is possible to disrupt a complex function such as language through damage to any of the sub-component processes.9 A useful analogy here is that of the car engine. The motion of the car is caused by the action of the engine, and this consists of a number of sub-component systems. It is possible to interfere with the engine’s ability to move the car through damage to any of these systems. A blocked fuel line would prevent fuel reaching the engine; faulty spark plugs would interfere with the ignition of fuel. Disruption of any of the sub-components would have the result that the car would cease to move. The function would be destroyed -but the function of the engine cannot be localized to just one site. Spark plugs are necessary for motion, but they are not the ‘centre’ or the site at which motion is controlled. The same situation holds for even more complex systems such as language. Damage to an area of the left temporal lobe may impair language ability – but the lost behaviour is not necessarily underpinned by that cortical site alone. It may be that the site was responsible for one, albeit vital, sub-component of the complex behaviour. Simple localization is unrealistic – the best we can hope for is to attempt to identify the location of the sub-component processes which operate together to produce the complex function.
The investigation of the localization of functions within a hemisphere uses a number of techniques. Lesion studies are common. After an individual has suffered a brain injury, the pattern of behavioural preservation and loss is documented and then the control of the lost behaviours is attributed to the site of the brain damage (i.e. the actual site that was deprived of a blood supply during a stroke, or the location of a tumour). In the past, the location of a lesion in a human subject was established at autopsy, but advances in neuroradiography permit identification of the lesion site soon after the onset of the condition, and in the living patient. Techniques used have included CT scanning (computerized tomography) and, more recently, MRI imaging. These techniques differ from those of functional brain imaging (p. 102) in that they record images of brain tissues and structure, and not the levels of activity within different brain regions. The new generation of functional brain imaging techniques suggest that the results of lesion studies, in which lost functions are attributed to the lesioned brain area, should be treated with some caution. Functional brain scans often reveal that the region of reduced brain metabolism which is the consequence of brain damage extends beyond the actual extent of the structural lesion. This reflects the connectivity of a brain region with other cortical sites: a lesion at one site can disrupt the flow of impulses to and from connected brain regions, with knock-on effects on the functions of the distant areas.
Lesion studies and the various methods of brain imaging have revealed that the regions of the cerebral hemispheres are specialized for different functions. For example, the anterior part of the frontal lobes, sometimes labelled the pre-frontal cortex, is involved in the planning and initiating of behaviour. The functions of the pre-frontal cortex are gathered together under the umbrella term of the executive function. The executive system (like an executive or manager in a business) is hypothesized to have an oversight of the activities of the individual. Other brain systems will control and organize behaviour in situations that are routine; but where the situation is novel, or routine operations have not been successful, the executive system will intervene and plan and control behaviour. For example, in routine language tasks such as naming pictures, functional brain scans reveal an increase in activity in the left superior temporal lobe, the boundary between the temporal and the parietal cortex, and in the movement control regions of cortex. If the word retrieval task is less routine, such as naming as many items as possible in a two-minute period from a category such as animals or words beginning with ‘f’, then there is an increase in pre-frontal cortex activity. Patients with pre-frontal damage display a range of personality and intellectual difficulties: aggression, antisocial behaviour and impulsiveness, under the former heading; poor memory, slow learning and impaired thinking, under the latter. A classic lesion study of the effects of pre-frontal cortex damage was reported in 1868 and concerned a railway construction worker, Phineas Gage. Following an explosion that drove a metal rod through his left cheek and up into the cranial cavity, damaging the left pre-frontal cortex, Gage’s personality was reported as changed. Although previous to the accident he had been a diligent worker, he became rude, uncaring of others, and constantly made plans which he never enacted. He was unable to keep a job and became an exhibit in a freak show in a travelling circus.
The functions of the pre-frontal cortex are complex, and faculties such as thinking and personality are difficult to study. The investigation of other functions is perhaps more straightforward. There are regions of cortex which receive sensory information and which are responsible for the first stages in the decoding and perception of the input. Visual information is relayed to the primary visual cortex in the occipital lobe, and lesions in this area can cause loss of visual perception, or abnormalities in various components of visual information such as colour, form and motion. Kinaesthetic information from a variety of skin and muscle receptors is processed in the parietal lobe in the region of the postcentral gyrus. This area is referred to as the primary’ somatosensory cortex and damage in this area causes loss of sensation (or hemianaesthesia) on the contralateral side of the body. The temporal lobe is important in the processing of auditory information, and the superior temporal gyrus is the location of the primary auditory cortex, the integrity of which is particularly important in the understanding of speech. The medial regions of the temporal lobe also contain structures which are important for the consolidation of new information into memory. These deep regions of the temporal lobe form the limbic system, and consist of structures such as the hippocampus, fornix and amygdala. Damage to these structures results in various forms of amnesia. The primary motor cortex is located in the posterior part of the frontal lobe, in the area of the precentral gyrus. This region is important in the control of movement. Lesions here will result in a loss of voluntary movement on the opposite side of the body. If both the upper and lower limbs are affected, this is labelled hemiplegia; if just one limb is involved, the disorder is labelled monoplegia. Involvement of all four limbs is quadriplegia.
Surrounding the areas of the primary motor cortex and the various forms of sensory cortex are very large areas of secondary or association cortex. These regions are involved in higher-order processing of information from the related primary cortex. The secondary motor cortex lies anterior to the primary motor cortex. Electrical stimulation of this region does not result in simple muscle twitches at the periphery; rather, complex and integrated patterns of movement occur – for example, the subject may reach out and grasp, and this region may be involved in the organization of speech movements. These plans are then relayed to the primary motor cortex for implementation. Lesions of this area do not cause paralysis of muscles; rather, movement is difficult to initiate and is poorly executed. This disorder is labelled apraxia (or dyspraxia, see p. 182).
Areas of secondary sensory cortex also lie near to all the cortical zones responsible for receiving incoming information. Electrical stimulation of these zones results in a subject reporting complex patterns of sensory experience, whereas stimulation of the primary sensory cortex results in simpler sensory experiences (for example, following stimulation of the secondary visual cortex, the subject might report complex hallucinations, whilst stimulation of the primary cortex results in perceptions such as flashes of light). The function of the secondary sensory areas is seen as one of perceiving, integrating and categorizing incoming information. Lesions in these areas typically result in patients reporting that, for example, they can see an object in the visual domain, but they are unable to recognize what it is. In the auditory domain, patients might report that they can hear speech, but it sounds like a foreign language. These disorders are labelled agnosias (see p. 219).
We can begin to see from this outline why large areas of the left hemisphere in particular are active during language processing. Sending a message involves decisions to initiate behaviour and the formation of a communicative plan. Sending messages – whether spoken or written – requires planning and implementing movements. Movement results in feedback from a range of sensory receptors – auditory, tactile and kinaesthetic. Receiving linguistic messages from others will involve either visual or auditory processing in both primary and secondary cortex. Then, in addition to brain regions involved in movement control and sensory processing, two brain regions have been claimed to be especially important in the encoding and decoding of linguistic messages. A number of researchers suggest that the anterior regions of the left hemisphere – areas within the frontal lobe in particular – are important in the formulation of messages, whilst regions of the temporal lobe are essential in message decoding. These ideas were established in the middle to late nineteenth century by the European neurologists Pierre Broca (1824-1880) and Karl Wernicke (1848-1905). Broca identified an area within the frontal lobe (the third frontal convolution), subsequently named after him as ‘Broca’s area’, which was important in the control of speech. Patients who suffered damage to this area were thought to have difficulties in formulating speech, but few difficulties in understanding messages. Wernicke identified an area of the temporal lobe (the first temporal convolution, or subsequently ‘Wernicke’s area’) which he suggested was important in the decoding of speech. Damage to this area resulted in fluent speech but great difficulties in the understanding of spoken messages.
These precise localizations of components of language processing have been debated ever since they were established. Hughlings Jackson (p. 57) was an early critic, and the debates continue to the present day. But as we increase our understanding of language as a complex higher cognitive function, and also our ability to record patterns of brain activation during language processing, our models of localization of language processing in the brain will become more sophisticated – recognizing the importance of large areas of the left and possibly also the right hemisphere, and even of subcortical structures. Other stages within the communication chain are capable of much more precise localization within the brain and it is to these we will now turn.
Speech and the Brain
It is evident from the discussion of localization of functions within the cortex that the brain areas involved in the control of movement and sensory feedback from skin and muscle receptors have been quite precisely localized. Control of voluntary movement by primary and secondary motor cortices is centered in the region of the cortex immediately anterior to the Rolandic fissure, and kinaesthetic information is processed by the primary and secondary sensory cortex in the area of the brain lying immediately posterior to the Rolandic fissure. One of the earliest findings of neurological investigations of the brain was that there was a stateable relationship between peripheral parts of the body (for example, the fingers) and parts of the primary motor and sensory cortices. Hence, electrical stimulation of a specific part of the motor or sensory cortex results in specific consequences at the periphery. Thus, if the area of the motor cortex which controls finger movement is stimulated, then the muscles of the fingers on the opposite side will twitch. The motor activities of the body are represented within the motor cortex in an ‘upside-down’ order: the uppermost part of the region seems to control the legs, and the lowest part the face. A common way of representing the relationship is to draw the ‘motor homunculus’ – an invention of the American neurologist, Wilder Graves Penfield.10 This is a figure of a human form in which the size of the parts of the body is made proportional to the extent of the brain area which is involved with them (Figure 4.6a). Notice the large area serving the muscles of the hand (especially the thumb), the face, the tongue, and the eye. Remember also that we have said that the motor cortex in each hemisphere controls the movements on the opposite side of the body. This is because the bundles (or ‘tracts’) of nerve fibres from the motor cortex, known as the pyramidal tracts, cross over each other on their way down through the brain stem, when they reach the medulla oblongata. It is this that accounts for the fact that brain damage to one hemisphere is correlated with effects, such as paralysis, on the contralateral side of the body. The sensory cortex shows a similar organization to the motor cortex, and a ‘sensory homunculus’ has likewise been drawn (Figure 4.6b). Notice here the large area devoted to the skin of the fingertips, the mouth area and tongue, and the tiny area given to the skin of the trunk. As with the motor cortex, the sensory cortex receives input from the opposite side of the body, so that damage to the left cortex results in sensory deficits on the right side of the body.
Figure 4.6. (a) Penfield’s motor homunculus; (b) Penfield’s sensory homunculus
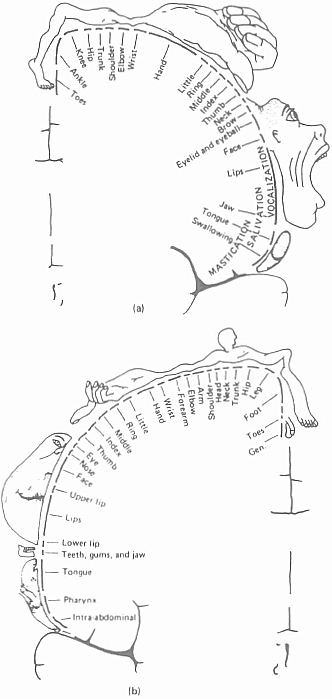
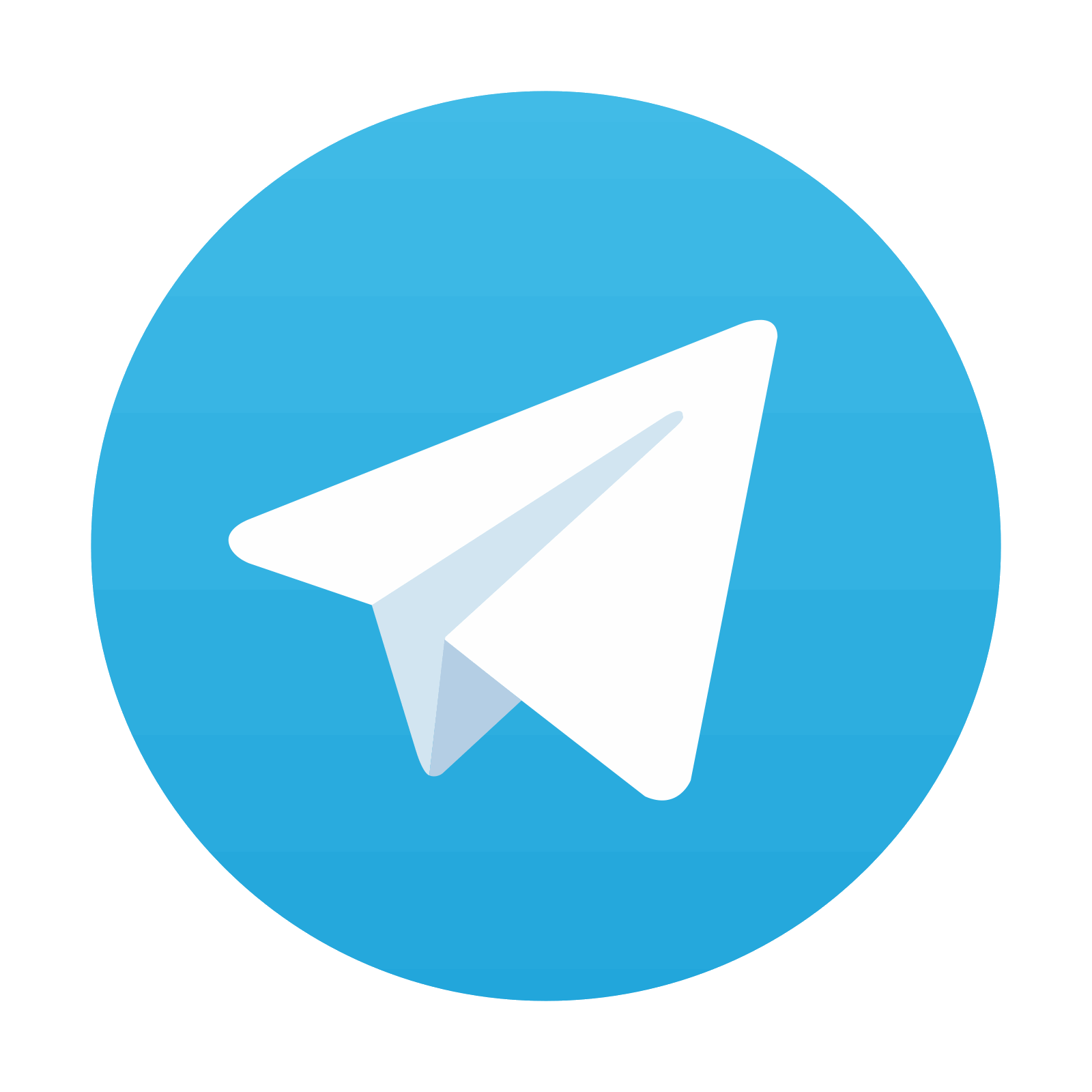
Stay updated, free articles. Join our Telegram channel
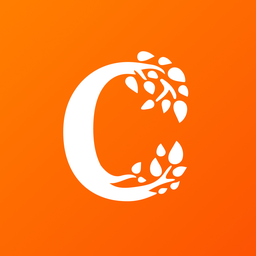
Full access? Get Clinical Tree
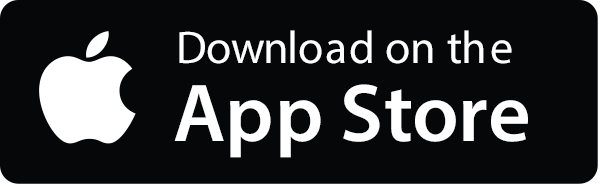
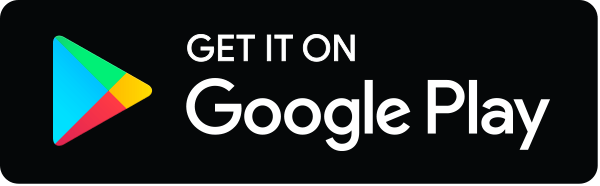