The Kidneys
In this chapter kidney function and how it can be altered in disease states is discussed. It is best read in conjunction with Chapters 2, 4 and 5. Interpretation of renal function tests is also discussed.
The kidneys excrete metabolic waste products, and have an essential homeostatic function in that they control the body solute and water status and the acid-base balance. There are about one million nephrons per kidney, each of which is made up of five main functional segments (Fig. 3.1).
The glomeruli, in the cortex of the kidney, are invaginated and surround a capillary network of blood vessels derived from the afferent, and draining into the efferent, arterioles. Small molecules and water are passively filtered during the passage of blood through these capillaries, the ultrafiltrate passing through the vessel walls and the glomerular membranes into the glomerular spaces (Bowman’s capsules).
The proximal convoluted tubules, also in the cortex, receive filtrate from the glomerular spaces. Convolution increases the tubular length and therefore contact between the luminal fluid and the proximal tubular cells.
The loops of Henle extend down into the renal medulla and ascend again after forming the loop.
The distal convoluted tubules, situated in the cortex, are important for fine adjustment of luminal fluid. They lie near the afferent arterioles, with the juxtaglomerular apparatus between them. The enzyme renin is produced by the latter and its release is controlled by local blood flow.
The collecting ducts start as the distal tubules lead down into the medulla and end by opening into the
renal pelvis. The modified fluid from the original filtrate flows from the collecting ducts into the renal tract.
renal pelvis. The modified fluid from the original filtrate flows from the collecting ducts into the renal tract.
Normal function of the kidneys depends on the following:
an adequate blood supply, which under normal circumstances is about 20 per cent of the cardiac output, flowing through the kidneys,
normal secretion and feedback control of hormones acting on the kidney,
the integrity of the glomeruli and the tubular cells.
In addition to the excretory function and acid- base control, the kidneys have important endocrine functions, including:
production of 1,25-dihydroxyvitamin D, the active metabolite of vitamin D, which is produced following hepatic hydroxylation of 25-hydroxyvitamin by the renal enzyme 1-hydroxylase,
production of erythropoietin, which stimulates erythropoiesis.
RENAL GLOMERULAR FUNCTION
About 200 L of plasma ultrafiltrate usually enter the tubular lumina daily, mainly by glomerular filtration into glomerular capsules but also through the spaces between cells lining the tubules (tight junctions). Production of ultrafiltrate depends on the blood flow through normal glomeruli and on the difference between the hydrostatic pressure gradient and the plasma effective colloid osmotic (oncotic) pressure gradient across the membranes (Fig. 3.2) and tight junctions. The colloid osmotic effect is weak relative to the hydrostatic gradient but does facilitate some reabsorption of fluid from the proximal renal tubules.
![]() Figure 3.2 The relationship between flow of blood through the glomerulus and the factors that affect the rate of filtration across the glomerular basement membrane. |
The filtrate contains diffusible constituents at almost the same concentrations as in plasma. About 30 000 mmol of sodium, 800 mmol of potassium, 800 mmol of urea, 300 mmol of free ionized calcium and 1000 mmol of glucose are filtered daily. Proteins (mainly low-molecular-weight proteins) and protein-bound substances are filtered in only small amounts by normal glomeruli and most are reabsorbed. The huge volume of filtrate allows adequate elimination of waste products such as urea; death from water and electrolyte depletion would occur within a few hours were the bulk of this water containing essential solutes not reclaimed.
RENAL TUBULAR FUNCTION
Changes in filtration rate alter the total amount of water and solute filtered, but not the composition of the filtrate. From the 200 L of plasma filtered daily, only about 2 L of urine are formed. The composition of urine differs markedly from that of plasma, and therefore of the filtrate. The tubular cells use adenosine triphosphate-dependent active transport, sometimes selectively, against physicochemical gradients. Transport of charged ions tends to produce an electrochemical gradient that inhibits further transport. This is minimized by two processes.
Isosmotic transport This occurs mainly in the proximal tubules and reclaims the bulk of filtered essential constituents. Active transport of one ion leads to passive movement of an ion of the opposite charge in the same direction, along the electrochemical gradient. The movement of sodium (Na+) depends on the availability of diffusible negatively charged ions, such as chloride (Cl−). The process is ‘isosmotic’ because the active transport of solute causes equivalent movement of water reabsorption in the same direction. Isosmotic transport also occurs to a lesser extent in the distal part of the nephron.
Ion exchange This occurs mainly in the more distal parts of the nephrons and is important for fine adjustment after bulk reabsorption has taken place. Ions of the same charge, usually cations, are exchanged and neither electrochemical nor osmotic gradients are created.
Therefore, during cation exchange there is insignificant net movement of anions or water. For example, Na+ may be reabsorbed in exchange for potassium (K+) or hydrogen (H+) ions. Na+ and H+ exchange also occurs proximally, but at that site it is more important for bicarbonate reclamation than for fine adjustment of solute reabsorption (see Chapter 4). In the cells lining the renal tubules, the intestine and
many secretory organs, the pumps are located on the membrane on one side of the cell only and therefore solute flows in one direction.
many secretory organs, the pumps are located on the membrane on one side of the cell only and therefore solute flows in one direction.
Other substances, such as phosphate and urate, are secreted into, as well as reabsorbed from, the tubular lumen. The tubular cells do not deal actively with waste products such as urea and creatinine to any significant degree. Most filtered urea is passed in urine (which accounts for most of the urine’s osmolality), but some diffuses back passively from the collecting ducts with water; by contrast, some creatinine is secreted into the tubular lumen.
Reclamation of solute from the proximal tubule
Almost all the potassium is actively reabsorbed from the proximal tubules, as is more than 70 per cent of the filtered sodium, free ionized calcium and magnesium. Some free ionized calcium is reabsorbed at more distal sites, possibly from the loops of Henle. This reabsorption may be stimulated by parathyroid hormone (PTH) and inhibited by loop diuretics such as furosemide. Only about 2 per cent of filtered calcium appears in the urine.
Many inorganic anions follow an electrochemical gradient; the reabsorption of sodium is limited by the availability of chloride, the most abundant diffusible anion in the filtrate. Bicarbonate is almost completely recovered following exchange of sodium and hydrogen ions (see Chapters 2 and 4). Specific active transport mechanisms result in the almost complete reabsorption of glucose, urate and amino acids. Some urate is secreted into the lumina, mainly in the proximal tubules, but most of this is reabsorbed.
Phosphate reabsorption is incomplete; phosphate in tubular fluid is important for buffering hydrogen ions. Inhibition of phosphate reabsorption by PTH occurs in both the proximal and the distal convoluted tubules, and accounts for the hypophosphataemia of PTH excess. Thus almost all the reusable nutrients and the bulk of electrolytes are reclaimed from the proximal tubules, with fine homeostatic adjustment taking place more distally. Almost all the filtered metabolic waste products, such as urea and creatinine, which cannot be reused by the body, remain in the luminal fluid.
WATER REABSORPTION: URINARY CONCENTRATION AND DILUTION
Water is always reabsorbed passively along an osmotic gradient. However, active solute transport is necessary to produce this gradient (see also Chapter 2). Two main processes are involved in water reabsorption:
Isosmotic reabsorption of water from the proximal tubules. The nephrons reabsorb 99 per cent of the filtered water, about 70-80 per cent (140-160 L/day) of which is returned to the body from the proximal tubules. Active solute reabsorption from the filtrate is accompanied by passive reabsorption of an osmotically equivalent amount of water. Therefore, fluid entering the lumina of the loops of Henle, although much reduced in volume, is still almost isosmotic.
Dissociation of water reabsorption from that of solute in the loops of Henle, distal tubules and collecting ducts. Normally between 40 and 60 L of water enter the loops of Henle daily. This volume is reduced to about 2 L as varying amounts of water are reabsorbed, helping to correct for changes in extracellular osmolality. At extremes of water intake, urinary osmolality can vary from about 40 to 1400 mmol/kg. The proximal tubules cannot dissociate water and solute reabsorption, and the adjustment must occur between the end of the proximal tubule and the end of the collecting duct.
Two mechanisms are involved:
Countercurrent multiplication is an active process occurring in the loops of Henle, whereby a high osmolality is created in the renal medulla and urinary osmolality is reduced. This can occur in the absence of antidiuretic hormone (ADH), also called arginine vasopressin or vasopressin, and a dilute hypo-osmolal urine is produced.
Countercurrent exchange is a passive process, occurring only in the presence of ADH. Water without solute is reabsorbed from the collecting ducts into the ascending vasa recta along the osmotic gradient created by countercurrent multiplication and by the high osmolality in the medulla, producing a concentrated urine.
Countercurrent multiplication
This occurs in the loops of Henle. It depends on the close apposition of the descending and ascending limbs of the loops to the vasa recta. The vasa recta make up a capillary network derived from the efferent arterioles and, like the loops of Henle, pass deep into the medulla.
The descending limbs are permeable to water but the thick ascending limbs are impermeable to water and solute. Chloride is actively pumped from the thick ascending to the descending limbs as fluid flows through the lumina of the loops; positively charged sodium ions follow along the electrochemical gradient. Thus, the osmolality progressively increases in the descending limbs and renal medullary interstitium; it decreases in the ascending limbs, but, as these are impermeable to water, this change is not transmitted to the interstitium.
The almost isosmolal fluid enters the descending limbs having the same osmolality as the plasma, just under 300 mmol/kg. If the fluid in the loops was stationary and no pumping had taken place, the osmolality throughout the loops and the adjacent medullary tissue would be about 300 mmol/kg (Fig. 3.3a).
![]() Figure 3.3 The renal counter-regulatory system. D, descending loop of Henle; A, ascending loop of Henle. |
Suppose the fluid column remained stationary and 1 mmol of solute per kilogram were pumped from the ascending into the descending limb, the result would be as in Figure 3.3b. If this pumping continued and there were no flow, the fluid in the descending limb would become hyperosmolal and that in the ascending limb correspondingly hypo-osmolal.
Suppose that the fluid flowed so that each figure ‘moved two places’ (Fig. 3.3c). As this happened, more solute would be pumped from the ascending to the descending limbs (Fig. 3.3d). If the fluid again flowed ‘two places’, the situation would be as shown in Figure 3.3e.
If these steps occurred simultaneously and continuously, the consequences would be as follows:
Increasing osmolality in the tips of the loops of Henle Because the walls of most of the loops are permeable to water and solute, osmotic equilibrium would be reached with the surrounding tissues in the deeper layers of the medulla, including the plasma within the vasa recta.
Countercurrent exchange (Fig. 3.4)
Countercurrent exchange is essential, together with multiplication, for regulating the osmolal concentration of urine. It can only occur in the presence of ADH and depends on the ‘random’ apposition of the collecting ducts and the ascending vasa recta. Antidiuretic hormone increases the permeability of the cell membranes (via the aquaporins) lining the distal parts of the collecting ducts to water, which then moves passively along the osmotic gradient created by multiplication. Consequently luminal fluid is concentrated as the collecting ducts pass into the increasingly hyperosmolal medulla.
![]() Figure 3.4 The countercurrent mechanism, showing the relationship between the renal tubules and the vasa recta. ADH, antidiuretic hormone. |
The increasing concentration of the fluid would reduce the osmotic gradient as it passes down the ducts if it did not meet even more concentrated plasma flowing in the opposite (countercurrent) direction. The gradient is thus maintained, and water continues to be reabsorbed until the fluid reaches the deepest layers, where the osmolality is about four or five times that of plasma (Fig. 3.3f). The low capillary hydrostatic pressure at this site and the osmotic effect of plasma proteins ensure that much of the reabsorbed water within the interstitium enters the vascular lumina. The diluted blood is carried towards the cortex and ultimately enters the general circulation and helps to dilute the extracellular fluid.
The osmotic action of urea in the medullary interstitium may potentiate the countercurrent multiplication. As water is reabsorbed from the collecting ducts under the influence of ADH, the luminal urea concentration increases. Because the distal collecting ducts are permeable to urea, it enters the
deeper layers of the medullary interstitium, increasing the osmolality and drawing water from the lower parts of the descending limbs of the loops. The amount of urea reabsorbed depends on:
deeper layers of the medullary interstitium, increasing the osmolality and drawing water from the lower parts of the descending limbs of the loops. The amount of urea reabsorbed depends on:
the amount filtered,
the rate of flow of tubular fluid: as much as 50 per cent of filtered urea may be reabsorbed when flow is significantly reduced.
In summary, both concentration and dilution of urine depend on active processes, which may be impaired if tubules are damaged.
Renal homeostatic control of water excretion
In this section, the mechanisms involved in the normal homeostatic control of urinary water excretion in the extremes of water intake are discussed. It may be helpful to read it in conjunction with Chapter 2, which deals with sodium and water balance.
Water restriction
By increasing the plasma osmolality, water restriction increases ADH secretion and allows countercurrent exchange with enhanced water reabsorption. Reduced circulatory volume results in a sluggish blood flow in the vasa recta and increased urea reabsorption, allowing a build-up of the medullary hyperosmolality produced by multiplication. This potentiates water reabsorption in the presence of ADH. The reduced capillary hydrostatic pressure and increased colloid osmotic pressure, due to the haemoconcentration following non-protein fluid loss, ensure that much of the reabsorbed water enters the vascular compartment.
Water load
A high water intake dilutes the extracellular fluid, and the consequent fall in plasma osmolality reduces ADH secretion. The walls of the collecting ducts therefore remain impermeable to water and the countercurrent multiplication produces a dilute urine and a high osmolality within the medulla and medullary vessels. Blood from the latter flows into the general circulation, so helping to correct the fall in systemic osmolality.
During maximal water diuresis the osmolality at the tips of the medullary loops may be 600 mmol/kg or less, rather than the maximum of about 1400 mmol/kg. Increasing the circulating volume increases renal blood flow; the rapid flow in the vasa recta ‘washes out’ medullary hyperosmolality, returning some of the solute, without extra water, to the circulation.
Thus, not only is more water than usual lost in the urine, more solute is ‘reclaimed’. Because medullary hyperosmolality, and therefore the ability to concentrate the urine maximally, is dependent on medullary blood flow, under normal circumstances urinary osmolality will be fully restored only several days after a prolonged water load has stopped (see Chapter 2).
Osmotic diuresis
An excess of filtered solute in the proximal tubular lumina impairs the bulk water reabsorption from this site by its osmotic effect. Unabsorbed solute concentration rises progressively as water is reabsorbed with other solute during passage through the proximal tubules, and this opposes further water reabsorption. Thus a larger volume than usual reaches the loops of Henle. Moreover, fluid leaving the proximal tubules, although still isosmotic with plasma, has a lower sodium concentration than plasma. The relative lack of the major cation (sodium) to accompany the anion chloride along the electrochemical gradient inhibits the pump in the loops. The resulting impairment of build-up of medullary osmolality inhibits distal water reabsorption, under the influence of ADH from the collecting ducts, resulting in a diuresis (see Chapter 2).
Normally most filtered water leaves the proximal tubular lumina with reabsorbed solute. For example, glucose (with an active transport system) and urea (which diffuses back passively) are sometimes filtered at high enough concentration to exceed the proximal tubular reabsorptive capacity. They can then act as osmotic diuretics and cause water depletion. This is important, for example, in diabetes mellitus or in uraemia.
The most effective osmotic diuretics are substances that cannot cross cell membranes to any significant degree; therefore, they must be infused, as they cannot be absorbed from the gut. One example is mannitol, a sugar alcohol, which is sometimes used therapeutically as a diuretic.
Homeostatic solute adjustment in the distal tubule and collecting duct
Sodium reabsorption in exchange for hydrogen ions occurs throughout the nephrons. In the proximal tubules the main effect of this exchange is on reclamation of filtered bicarbonate. In the distal tubules and collecting ducts, the exchange process is usually associated with net generation of bicarbonate to replace that lost in extracellular buffering. Potassium
and hydrogen ions compete for secretion in exchange for sodium ions. The possible mechanism stimulated by aldosterone is discussed in Chapter 2. The most important stimulus to aldosterone secretion is mediated by the effect of renal blood flow on the release of renin from the juxtaglomerular apparatus; this method of reabsorption is part of the homeostatic mechanism controlling sodium and water balance.
and hydrogen ions compete for secretion in exchange for sodium ions. The possible mechanism stimulated by aldosterone is discussed in Chapter 2. The most important stimulus to aldosterone secretion is mediated by the effect of renal blood flow on the release of renin from the juxtaglomerular apparatus; this method of reabsorption is part of the homeostatic mechanism controlling sodium and water balance.
BIOCHEMISTRY OF RENAL DISORDERS
Pathophysiology
Different parts of the nephrons are in close anatomical association and are dependent on a common blood supply. Renal dysfunction of any kind affects all parts of the nephrons to some extent, although sometimes either glomerular or tubular dysfunction is predominant. The net effect of renal disease on plasma and urine depends on the proportion of glomeruli to tubules affected and on the number of nephrons involved.
To understand the consequences of renal disease it may be useful to consider the hypothetical individual nephrons, first with a low glomerular filtration rate (GFR) and normal tubular function, and then with tubular damage but a normal GFR. It should be emphasized that these are hypothetical examples, as in clinical reality a combination of varying degree may exist.
Uraemia is the term used to describe a raised plasma urea concentration and is almost always accompanied by an elevated creatinine concentration: in North America this is usually referred to as azotaemia (a raised nitrogen concentration).
Reduced glomerular filtration rate with normal tubular function
The total amounts of urea and creatinine excreted are affected by the GFR. If the rate of filtration fails to balance that of production, plasma concentrations will rise.
Phosphate and urate are released during cell breakdown. Plasma concentrations rise because less than normal is filtered. Most of the reduced amount reaching the proximal tubule can be reabsorbed, and the capacity for secretion is impaired if the filtered volume is too low to accept the ions; these factors further contribute to high plasma concentrations.
A large proportion of the reduced amount of filtered sodium is reabsorbed by isosmotic mechanisms; less than usual is then available for exchange with hydrogen and potassium ions distally. This has two main outcomes:
reduced hydrogen ion secretion throughout the nephron: bicarbonate can be reclaimed only if hydrogen ions are secreted; plasma bicarbonate concentrations will fall,
reduced potassium secretion in the distal tubule, with potassium retention (potassium can still be reabsorbed proximally).
If there is a low GFR accompanied by a low renal blood flow:
Systemic aldosterone secretion will be maximal: in such cases, any sodium reaching the distal tubule will be almost completely reabsorbed in exchange for H+ and K+, and the urinary sodium concentration will be low.
ADH secretion will be increased: ADH acting on the collecting ducts allows water to be reabsorbed in excess of solute, further reducing urinary volume and increasing urinary osmolality well above that of plasma and reducing plasma sodium concentration. This high urinary osmolality is mainly due to substances not actively dealt with by the tubules. For example, the urinary urea concentration will be well above that of plasma. This distal response will occur only in the presence of ADH; in its absence, normal nephrons will form a dilute urine.
If the capacity of the proximal tubular cells to reabsorb solute, and therefore water, is normal, a larger proportion than usual of the reduced filtered volume will be reclaimed by isosmotic processes, thus further reducing urinary volume.
In summary, the findings in venous plasma and urine from the affected nephrons will be as follows.
Plasma
High urea (uraemia) and creatinine concentrations.
Low bicarbonate concentration, with low pH (acidosis).
Hyperkalaemia.
Hyperuricaemia and hyperphosphataemia.
Urine
Reduced volume (oliguria).
Low (appropriate) sodium concentration – only if renal blood flow is low, stimulating aldosterone secretion.
High (appropriate) urea concentration and therefore a high osmolality – only if ADH secretion is stimulated.
Reduced tubular function with normal glomerular filtration rate
Damage to tubular cells impairs adjustment of the composition and volume of the urine. Impaired solute reabsorption from proximal tubules reduces isosmotic water reabsorption. Countercurrent multiplication may also be affected, and therefore the ability of the collecting ducts to respond to ADH is reduced. A large volume of inappropriately dilute urine is produced.
The tubules cannot secrete hydrogen ions and therefore cannot reabsorb bicarbonate normally or acidify the urine. The response to aldosterone and therefore the exchange mechanisms involving reabsorption of sodium are impaired; the urine contains an inappropriately high concentration of sodium for the renal blood flow. Potassium reabsorption from the proximal tubule is impaired and plasma potassium concentrations may be low. Reabsorption of glucose, phosphate, magnesium, urate and amino acids is impaired. Plasma phosphate, magnesium and urate concentrations may be low.
Thus, the findings in venous plasma and urine from the affected nephrons will be as follows.
Plasma
Normal urea and creatinine concentrations (normal glomerular function).
Due to proximal or distal tubular failure:
low bicarbonate concentration and low pH,
hypokalaemia.
Due to proximal tubular failure:
hypophosphataemia, hypomagnesaemia and hypouricaemia.
Urine
Due to proximal and/or distal tubular failure:
increased volume,
pH inappropriately high compared with that in plasma.
Due to proximal tubular failure:
generalized amino aciduria,
phosphaturia,
glycosuria.
Due to distal tubular failure:
even if renal blood flow is low, an inappropriately high sodium concentration (inability to respond to aldosterone),
There may also be tubular proteinuria, which usually refers to low-molecular-weight proteins that are normally produced in the body, filtered across the glomerular membrane and reabsorbed in the proximal tubule, but appear in the urine as a result of proximal tubular damage, for example α1-microglobulin and retinol binding protein. However, tubular proteinuria also occurs when proximal tubular enzymes and proteins, such as N-acetyl-β-D-glucosaminidase (NAG), are released into the urine due to tubular cell injury. See Chapter 19.
Clinical and biochemical features of renal disease
The biochemical findings and urine output in renal disease depend on the relative contributions of glomerular and tubular dysfunction. When the GFR falls, substances that are little affected by tubular action (such as urea and creatinine) are retained. Although their plasma concentrations start rising above the baseline for that individual soon after the GFR falls, they seldom rise above the reference range for the population until the GFR is below about 60 per cent of normal, although in individual patients they do rise above baseline.
Plasma concentrations of urea and creatinine depend largely on glomerular function (Fig. 3.5). By contrast, urinary concentrations depend almost entirely on tubular function. However little is filtered at the glomeruli, the concentrations of substances in the initial filtrate are those of a plasma ultrafiltrate. Any difference between these concentrations and those in the urine is due to tubular activity. The more the tubular function is impaired, the nearer the plasma concentrations will be to those of urine. Urinary concentrations inappropriate to the state of hydration suggest tubular damage, whatever the degree of glomerular dysfunction.
The plasma sodium concentration is not primarily affected by renal disease. The urinary volume depends on the balance between the volume filtered and the proportion reabsorbed by the tubules. As 99 per cent of filtered water is normally reabsorbed, a very small impairment of reabsorption causes a large increase in urine volume. Consequently, if tubular dysfunction predominates, impairment of water reabsorption causes polyuria, even though glomerular filtration is reduced (see Chapter 2).
The degree of potassium, phosphate and urate retention depends on the balance between the degree of glomerular retention and the loss as a result of a reduced
proximal tubular reabsorptive capacity. If glomerular dysfunction predominates, so little is filtered that plasma concentrations rise, despite the failure of reabsorption. Conversely, if tubular dysfunction predominates, glomerular retention is more than balanced by impaired reabsorption of filtered potassium, urate and phosphate, and therefore plasma concentrations may be normal or even low. A low plasma bicarbonate concentration is found in association with metabolic acidosis, which may worsen the hyperkalaemia.
proximal tubular reabsorptive capacity. If glomerular dysfunction predominates, so little is filtered that plasma concentrations rise, despite the failure of reabsorption. Conversely, if tubular dysfunction predominates, glomerular retention is more than balanced by impaired reabsorption of filtered potassium, urate and phosphate, and therefore plasma concentrations may be normal or even low. A low plasma bicarbonate concentration is found in association with metabolic acidosis, which may worsen the hyperkalaemia.
Acute kidney injury
This was previously known as acute renal failure. In adults, oliguria is defined as a urine output of less than 400 mL/day, or less than 15 mL/h; it usually indicates a low GFR and a rapid decline in renal function over hours to weeks, with retention of creatinine and nitrogenous waste products. Oliguria may be caused by the factors discussed below.
Acute oliguria with reduced GFR (pre-renal)
This is caused by factors that reduce the hydrostatic pressure gradient between the renal capillaries and the tubular lumen. A low intracapillary pressure is the
most common cause. It is known as renal circulatory insufficiency (‘pre-renal uraemia’) and may be due to:
most common cause. It is known as renal circulatory insufficiency (‘pre-renal uraemia’) and may be due to:
CASE 1
A 17-year-old man was involved in a road traffic accident. Both femurs were fractured and his spleen was ruptured. Two days after surgery and transfusion of 16 units of blood, the following results were found:
Plasma
Sodium 136 mmol/L (135-145)
Potassium 6.1 mmol/L (3.5-5.0)
Urea 20.9 mmol/L (2.5-7.0)
Creatinine 190 µmol/L (70-110)
Albumin-adjusted calcium 2.40 mmol/L (2.15-2.55)
Phosphate 2.8 mmol/L (0.80-1.35)
Bicarbonate 17 mmol/L (24-32)
The patient was producing only 10 mL of urine per hour and a spot urinary sodium was 8 mmol/L.
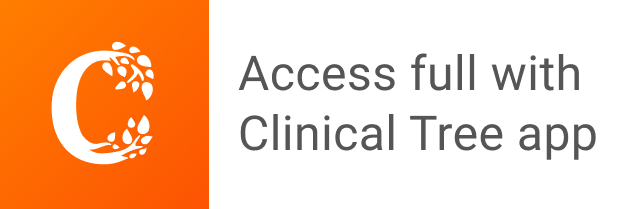