The Genitourinary System
Although the kidney is sometimes thought of as simply being an organ for waste elimination, it is much more than that. Major functions of the kidney include:
Regulation of fluid and electrolyte balance.
Maintenance of pH balance.
Regulation of calcium/phosphate balance.
Activation of intestinally absorbed vitamin D.
Excretion of waste products.
Regulation of blood pressure.
Production of erythropoietin.
● Physiologic Concepts
STRUCTURE
The kidneys lie outside the peritoneal cavity in the upper posterior portion of the abdominal wall, one on each side of the body. Each kidney is made up of approximately one million functional units, each of which is called a nephron. As shown in Figure 18-1, the nephron begins as a capillary tuft, called the glomerulus. Plasma is filtered across the glomerulus by the process of bulk flow and enters the twisting, looping tubule of the nephron. Of the plasma that enters the tubule, only a small fraction is excreted as urine. The remaining
plasma, compared with what entered the tubule across the glomerular capillary, has its final composition and volume drastically altered by the processes of renal reabsorption and secretion.
plasma, compared with what entered the tubule across the glomerular capillary, has its final composition and volume drastically altered by the processes of renal reabsorption and secretion.
![]() FIGURE 18-1 Structure of the nephron. (From Bullock, B.A., & Henze, R.L. (2000). Focus on pathophysiology. Philadelphia, PA: Lippincott Williams & Wilkins.) |
Each kidney is divided anatomically into an outer cortex containing all the glomerular capillaries and some short tubular segments, and an inner medulla where most of the tubular segments are located. The progression of tubular segments from the glomerulus to the proximal tubule, to the distal tubule, and finally to the collecting tubule is shown in Figure 18-1. Each nephron’s collecting tubule joins other collecting tubules to become several hundred large collecting ducts. The large collecting ducts are located in the renal papillae, which are located in the innermost portion of the kidney, the renal medulla.
The large collecting ducts feed into a central draining area, called the renal pelvis, and from there empty into the ureter. The ureter from each kidney is connected to the bladder (Fig. 18-2). The bladder stores urine until it is released outside the body by the process of micturition (urination). Micturition occurs through a single tube called the urethra.
The large collecting ducts feed into a central draining area, called the renal pelvis, and from there empty into the ureter. The ureter from each kidney is connected to the bladder (Fig. 18-2). The bladder stores urine until it is released outside the body by the process of micturition (urination). Micturition occurs through a single tube called the urethra.
RENAL BLOOD FLOW
The kidneys receive approximately 1000 to 1200 mL of blood per minute which is 20% to 25% of the cardiac output. Of that amount, only 1% to 2% goes to the medulla while the remainder circulates through the cortex. This high rate of blood flow is not required for meeting extraordinary energy demands, but for allowing the kidney to adjust the blood composition continually. By adjusting the blood composition, the kidney is able to maintain blood volume; ensure sodium, chloride, potassium, calcium, phosphate, and pH balance; and eliminate products of metabolism such as urea and creatinine.
Blood flows to the kidneys via the renal arteries, one renal artery to each kidney. In the kidney, the renal artery branches many times, ending as several afferent arterioles. Each afferent arteriole becomes the glomerular capillary that supplies a nephron with blood.
The glomerular capillary reforms not to become a venule as most capillaries do, but to form the efferent arteriole. This is shown in Figure 18-1. The efferent arteriole soon branches into a second capillary network, the peritubular capillaries, which surround and support the nephron tubules themselves. At the end of each nephron, the peritubular capillaries finally reform to venules. The
venules join to become veins. Blood leaves the kidney and heads back to the vena cava to be recirculated. The peritubular capillaries surrounding the long loop of the nephron (the loop of Henle) are called the vasa recta.
venules join to become veins. Blood leaves the kidney and heads back to the vena cava to be recirculated. The peritubular capillaries surrounding the long loop of the nephron (the loop of Henle) are called the vasa recta.
FILTRATION, REABSORPTION, AND SECRETION
Filtration refers to the bulk flow of plasma across the glomerular capillary into the interstitial fluid space surrounding the start of the nephron, an area called the Bowman space. At the glomerulus, approximately 20% of the plasma is continually filtered into the Bowman space. This filtrate is of the same composition as the plasma, except that protein molecules are not usually filtered. The initial filtrate diffuses across the Bowman space and into the beginning section of the tubule, Bowman capsule, to begin its journey through the rest of the tubule.
Most of the substances that enter the tubule at the Bowman capsule do not remain in the tubule. Instead, they move (or are moved) back into the blood across the peritubular capillaries by the process of reabsorption. Other substances are added to the urine filtrate, also across the peritubular capillaries, by the process of secretion. It is by reabsorption and secretion that the nephrons manipulate the composition and volume of the initial urine filtrate to produce the final urine.
GLOMERULAR FILTRATION
Glomerular filtration is the process by which approximately 20% of the plasma entering the glomerular capillary moves across the capillary into the interstitial space and from there into the Bowman capsule. Neither red blood cells nor plasma proteins are more than minimally filtered in healthy kidneys.
The process of filtration across the glomerulus is similar to that which occurs across all capillaries, as described in Chapter 13. What is different in the kidney is that the glomerular capillaries have increased permeability to small solutes and water. Also, unlike other capillaries, the forces favoring filtration of plasma across the glomerular capillary into the Bowman space are greater than the forces favoring reabsorption of fluid back into the capillary. Therefore, net filtration of fluid into the Bowman space occurs. This fluid then diffuses into the Bowman capsule and begins its journey through the rest of the nephron.
In the glomerulus, the primary force favoring filtration is capillary pressure. In most other capillaries, this pressure averages 18 mm Hg; in the glomerulus the average pressure is almost 60 mm Hg. This higher capillary pressure occurs as a result of decreased resistance to flow offered by the afferent arteriole feeding the glomerulus, compared with arterioles elsewhere. Therefore, the hydrostatic pressure reaching the glomerulus is greater, as shown in Figure 18-3.
Interstitial fluid pressure in the Bowman space is also much greater than in normal interstitial spaces (approximately 15 mm Hg vs. approximately −3 mm Hg). This greater pressure is a result of the high fluid volume entering
the Bowman space from the glomerulus, thus opposing further glomerular filtration. Capillary concentration of protein (plasma colloid osmotic pressure) is the same in the glomerulus as in other capillaries. The plasma colloid osmotic pressure increases throughout the length of the glomerulus as the protein-free filtrate is pushed into the Bowman space, averaging approximately 28 mm Hg overall; this force opposes glomerular filtration. The interstitial fluid colloid osmotic pressure (the pressure exerted by interstitial proteins) is normally approximately 8 mm Hg; this pressure favors glomerular filtration.
the Bowman space from the glomerulus, thus opposing further glomerular filtration. Capillary concentration of protein (plasma colloid osmotic pressure) is the same in the glomerulus as in other capillaries. The plasma colloid osmotic pressure increases throughout the length of the glomerulus as the protein-free filtrate is pushed into the Bowman space, averaging approximately 28 mm Hg overall; this force opposes glomerular filtration. The interstitial fluid colloid osmotic pressure (the pressure exerted by interstitial proteins) is normally approximately 8 mm Hg; this pressure favors glomerular filtration.
Adding up the forces favoring filtration across the glomerulus (60 + 8 mm Hg) and the forces favoring reabsorption (28 + 15 mm Hg), a net force of approximately 25 mm Hg results favoring the filtration of plasma into the Bowman space. This filtrate enters the Bowman capsule, moves through the tubule, and a portion of it becomes urine.
Glomerular Filtration Rate
The glomerular filtration rate (GFR) is defined as the volume of filtrate entering the Bowman capsule per unit of time. GFR is nearly constant and gives a good indication of the health of the kidneys. GFR depends on the four forces determining filtration and reabsorption (capillary pressure, interstitial fluid pressure, plasma colloid osmotic pressure, and interstitial fluid colloid osmotic pressure). Therefore, any change in these forces can alter GFR. Likewise, GFR depends on the available surface area of the glomerulus for filtration. Therefore, a loss of glomerular surface area decreases GFR.
An average value for GFR in an adult is 180 L/day (125 mL/min). A normal plasma volume is approximately 3 L (out of a total blood volume of approximately 5 L). This means that the kidney filters the plasma approximately 60 times each day! Equally remarkable is the fact that of the 180 L/day filtered into the Bowman capsule, only approximately 1.5 L/day are excreted from the body as urine. The rest is reabsorbed back into the blood across the peritubular capillaries.
Measurement of Glomerular Filtration Rate
GFR measurement is possible if one has a substance (call it x) that is freely filterable at the glomerulus and then is not reabsorbed, secreted, or changed in any way before it appears in the urine. To calculate the GFR from this substance, one would measure its concentration in a plasma sample (Px), its concentration in a urine sample (Ux), and the urine volume over a certain period of time (V). Given these values, the equation for GFR, in milliliters per minute, can be solved as shown in the following equation:

The classic substance that fits the criteria described above for substance x is the polysaccharide inulin. However, inulin is not normally present in the body, which means using inulin to measure GFR involves infusing it into an individual for an extended period. This offers a highly accurate but impractical method for measuring GFR. Instead, what is usually measured in plasma and urine is the concentration of creatinine, which is a naturally produced protein.
Creatinine is produced as a result of normal daily protein metabolism, a process that is assumed to occur at a nearly constant rate. This assumption may not hold true, for example, it may increase after muscle trauma or intense exercise. To measure GFR using creatinine, a blood sample is drawn along with a timed urine sample, and creatinine concentrations in the blood and urine are measured.
GFR measured from creatinine concentration and urine volume is only an estimate of the true GFR, because a small amount of creatinine is actually secreted into the lumen of the tubule from the peritubular capillaries. Therefore, GFR estimated by creatinine will be slightly high, because more creatinine will
be excreted in the urine than was filtered at the glomerulus. Measurement of GFR is important because it offers a clue to nephron function. In conditions of disease leading to renal failure, GFR falls.
be excreted in the urine than was filtered at the glomerulus. Measurement of GFR is important because it offers a clue to nephron function. In conditions of disease leading to renal failure, GFR falls.
RENAL CLEARANCE
The concentration of a substance totally cleared from the blood into the urine over time is known as the renal clearance. The GFR described above for inulin is actually the clearance of inulin, because all filtered inulin is cleared by the kidneys (it is neither reabsorbed nor secreted). For creatinine, clearance is actually slightly greater than the GFR, because some creatinine is secreted into the urine as well as filtered.
Other substances not normally excreted in the urine, such as glucose, have zero clearance. Although glucose is freely filtered across the glomerulus, it is normally totally reabsorbed by the tubules and none appears in the urine (i.e., none is cleared). Substances that are partially reabsorbed back into the plasma, for example, sodium and chloride ion, are cleared at a rate less than the GFR but greater than zero. Substances that are secreted from the blood into the tubule are cleared at a rate greater than the GFR.
Measuring the clearance of any substance is done by the same technique as measuring GFR. The concentration of the substance in the plasma and urine is determined, as is the urine volume over a given period. The equation expressing the clearance of any substance is UV/P, where U is the concentration of the substance in the urine (milligrams [mg] per milliliter [mL]), V is the volume per time of urine (mg/mL), and P is the concentration of the substance in the plasma (mg/mL).
Only for a substance like inulin (freely filtered, neither reabsorbed nor secreted) is the GFR equal to the clearance. For all other substances, clearance is either more or less than the GFR. Measuring the clearance of a plasma substance that is 100% excreted by the kidneys allows one to estimate renal plasma flow, and from there, renal blood flow.
MEASUREMENT OF RENAL PLASMA FLOW AND RENAL BLOOD FLOW
Measuring renal plasma flow usually involves measuring the clearance of a substance called para-aminohippurate (PAH). PAH is freely filtered at the glomerulus. It is not reabsorbed, but is actively secreted into the urine filtrate. Therefore, all PAH in the plasma (100%) is cleared by the kidneys. The clearance of PAH gives an estimate of renal plasma flow. Because the plasma is approximately 40% to 50% of the total blood volume, this allows one to estimate renal blood flow.
It is only possible to estimate renal blood flow from clearance of PAH because not all plasma entering the kidney goes through a glomerular capillary. Approximately 10% to 15% of renal blood flow feeds nonfiltering tissue such
as renal fat and connective tissue. Therefore, clearance of PAH is said to give the effective renal plasma flow (ERPF), which is 10% to 15% less than the total renal plasma flow, as shown in the following equation:
as renal fat and connective tissue. Therefore, clearance of PAH is said to give the effective renal plasma flow (ERPF), which is 10% to 15% less than the total renal plasma flow, as shown in the following equation:

From the ERPF, the effective renal blood flow (ERBF) can be found with the following equation:

where Vc is the measured hematocrit of the blood sample (the amount of blood occupied by red blood cells, not plasma).
REGULATION OF RENAL BLOOD FLOW
Maintenance of adequate renal blood flow is essential for kidney survival and for control of plasma volume and electrolytes. Changes in renal blood flow may increase or decrease the glomerular hydrostatic pressure, affecting GFR. The kidney has several mechanisms for controlling renal blood flow. These mechanisms serve to maintain both kidney function and GFR constant in spite of systemic blood pressure changes.
Renal blood flow is controlled by intrarenal and extrarenal mechanisms. Intrarenal mechanisms include the inherent ability of the afferent and the efferent arterioles to dilate or constrict, thereby controlling blood flow through the kidney. This inherent ability is called autoregulation. Extrarenal mechanisms regulating renal blood flow include the direct effects of increased or decreased mean arterial pressure and the effects of the sympathetic nervous system. A third mechanism regulating renal blood flow that has both intrarenal and extrarenal components involves a hormone produced by the kidney that affects the entire systemic circulation. This hormone, called renin, exerts its effects through the production of a potent vasoconstrictor, angiotensin II (AII).
Autoregulation
Autoregulation is the intrinsic response of vascular smooth muscle to changes in blood pressure. Like many arterioles, smooth muscle cells of the afferent and the efferent arterioles respond to their own stretch with reflex constriction. When systemic blood pressure is increased, stretch on afferent arterioles is increased. Stretching the afferent arterioles causes them to constrict, reducing the blood flow and returning renal blood pressure back toward normal. In contrast, when systemic blood pressure is decreased, stretch on the afferent and the efferent arterioles is reduced, and the arterioles respond by relaxing and
dilating to increase flow. As a result of autoregulation, renal blood flow remains nearly constant over a range of blood pressures between 80 and 180 mm Hg.
dilating to increase flow. As a result of autoregulation, renal blood flow remains nearly constant over a range of blood pressures between 80 and 180 mm Hg.
Autoregulation is especially effective during blood pressure increases. The bottom limit of autoregulation, 80 mm Hg, however, is reached more frequently than the upper limit. Therefore, GFR may decrease with severe hypotension.
Sympathetic Nervous System
Sympathetic nerves innervate both the afferent and the efferent arterioles of the kidney and can override autoregulation when stimulated. As is true in most arterioles, stimulation of the sympathetic nerves causes constriction of the afferent arterioles, leading to increased resistance to flow. As a result, blood flow through the glomerulus decreases, causing a decrease both in capillary hydrostatic pressure and in GFR. Simultaneous sympathetic stimulation of the efferent arterioles, however, and their subsequent constriction, causes blood flow to back up in the glomerulus. This backup can actually increase capillary hydrostatic pressure and glomerular filtration. The net result of sympathetic stimulation to the kidneys is a significant decrease in renal blood flow (because blood going both in and out is reduced) but a lesser decrease in GFR. The sympathetic nervous system is stimulated when there is a decrease in systemic blood pressure.
Decreased renal blood flow in response to decreased systemic blood pressure is adaptive and helps the organism survive a hypotensive crisis. With hypotension, less water and salt are filtered at the glomerulus, causing less to be lost in the urine. This helps to increase blood volume and restore blood pressure.
In conditions of increased blood pressure, sympathetic stimulation to all arterioles is reduced. The afferent and the efferent arterioles dilate, and renal blood flow and GFR both increase. This change results in increased loss of water and salt in the urine, which helps to reduce blood volume and return blood pressure toward normal.
Note that sympathetic input dominates over autoregulatory mechanisms of the kidney. If sympathetic stimulation increases, renal blood flow decreases despite attempts by the kidney to autoregulate its flow.
Renin
Renin is a hormone released from the kidney in response to either a decrease in blood pressure or a decrease in plasma sodium concentration. Cells that synthesize and secrete renin and control its release are a particular group of cells of the nephron called the juxtaglomerular (JG) apparatus. This group of cells includes smooth muscle cells of the afferent arteriole and cells of the macula densa. The smooth muscle cells synthesize renin and act as baroreceptors monitoring blood pressure. Macula densa cells are part of the thick ascending limb of the nephron. These cells sense plasma sodium concentration. The macula densa cells and the afferent arteriolar cells are in close approximation
to each other where the ascending limb of the distal tubule nearly touches the glomerulus. When the macula densa cells sense a change in plasma sodium, they pass that message on to the renin-secreting cells.
to each other where the ascending limb of the distal tubule nearly touches the glomerulus. When the macula densa cells sense a change in plasma sodium, they pass that message on to the renin-secreting cells.
When blood pressure falls, the smooth muscle cells increase renin release. When blood pressure increases, the smooth muscle cells decrease their release of renin. If plasma sodium levels decrease, macula densa cells signal the reninproducing cells to increase their activity. If plasma sodium levels increase, macula densa cells signal the smooth muscle cells to decrease renin release.
Sympathetic nerves also stimulate the JG apparatus to secrete renin. Thus, decreased blood pressure causes increased renin both directly, via the JG baroreceptors, and indirectly, via the sympathetic nerves.
Once released, renin circulates in the blood and acts to catalyze the breakdown of a small protein, angiotensinogen, to a 10-amino-acid protein, angiotensin I (AI). Angiotensinogen is produced by the liver and is highly concentrated in the blood. Renin release is thus the rate-limiting step in the reaction. The conversion of angiotensinogen to AI occurs throughout the plasma, but primarily in the pulmonary capillaries. AI has few effects of its own, but it is quickly acted upon by another enzyme readily available in the bloodstream—angiotensin-converting enzyme (ACE). ACE splits AI into an 8-amino-acid peptide, angiotensin II (AII).
Angiotensin II
AII is a potent vasoconstrictor that acts throughout the vascular system to increase smooth muscle contraction, thereby decreasing vessel diameter and increasing total peripheral resistance (TPR). An increase in TPR directly increases systemic blood pressure (see Chapter 13). AII is also a potent hormone that circulates in the blood to the adrenal glands, causing the synthesis of the mineralocorticoid hormone aldosterone.
Aldosterone
Aldosterone circulates in the blood and binds to cells of the cortical collecting duct. The binding of aldosterone increases sodium reabsorption from the urine filtrate, causing sodium to return into the peritubular capillaries. Since water often follows sodium movement, increased sodium reabsorption allows for increased water reabsorption, causing increased plasma volume. An increase in plasma volume increases venous return to the heart, thereby increasing the stroke volume and cardiac output. Increased cardiac output, like increased TPR, directly increases systemic blood pressure.
Other stimuli for aldosterone release, besides AII, are high plasma potassium level and a hormone from the anterior pituitary, adrenocorticotropic hormone (ACTH). In addition to affecting sodium reabsorption, aldosterone stimulates the secretion (and therefore the excretion) of potassium from the cortical collecting duct into the urine filtrate. Aldosterone affects sodium and potassium transport across the gut, in the same manner as it does across the collecting duct.
Renin-Angiotensin Reflex Response to Changes in Blood Pressure
With a decrease in blood pressure, the JG cells release renin, which in turn causes an increase in AII. AII constricts arterioles throughout the body, including the afferent and the efferent arterioles. AII-induced constriction increases TPR and a return of blood pressure back toward normal (Fig. 18-4). Renal blood flow is reduced, which causes less urine to be produced. Decreased urine output contributes to increased plasma volume and blood pressure.
The opposite occurs with increased blood pressure. With an increase in blood pressure, renin release decreases, as do AII levels. This leads to dilation of systemic arterioles, a reduction of TPR, and a return of blood pressure back toward normal. Decreased AII causes afferent and efferent arterioles to relax, leading to an increase in renal blood flow and urine output, which also serves to decrease blood pressure.
Renin-Angiotensin-Aldosterone Response to Decreased Sodium
The second stimulus for renin release is plasma sodium concentration. Decreased sodium in the tubular fluid passing the cells of the macula densa causes increased
renin release. As shown in Figure 18-5, increased renin leads to increased AII, which stimulates aldosterone synthesis and therefore increases sodium reabsorption. Increased sodium reabsorption reduces the stimulus for further renin release. The opposite is true if there is increased plasma sodium passing the macula densa cells.
renin release. As shown in Figure 18-5, increased renin leads to increased AII, which stimulates aldosterone synthesis and therefore increases sodium reabsorption. Increased sodium reabsorption reduces the stimulus for further renin release. The opposite is true if there is increased plasma sodium passing the macula densa cells.
RENAL REABSORPTION
Reabsorption is the second process by which the kidney determines the concentration of a substance filtered from the plasma. Reabsorption refers to the active (requiring energy and always being mediated by a carrier) or the passive (no energy required) movement of a substance filtered at the glomerulus back into the peritubular capillaries. Reabsorption may be total (e.g., glucose) or partial (e.g., sodium, urea, chloride, and water).
Reabsorption of Glucose
Glucose is freely filtered at the glomerulus. All of the filtered glucose is normally reabsorbed by active transport, primarily in the proximal tubule.
Because carriers are involved, a transport maximum (Tm) for glucose can be reached. The Tm is the amount of a substance that can be transported per
unit of time. For glucose, at a certain filtered load (GFR × plasma concentration), all carriers become occupied. Any glucose filtered beyond that load is not reabsorbed, but is instead excreted in the urine. The Tm for glucose is approximately 375 mg/min of filtered glucose. The concentration of glucose which results in this filtered load, given a GFR of 125 mL/min, is 3.0 mg/mL of plasma because glucose concentration clinically is frequently expressed as per 100 mL of blood, or, 300 mg/dL. However, glucose begins to appear in the urine even before this plasma level is reached, because each nephron has a slightly different Tm and the carrier transport rate may accelerate at the highest glucose concentrations. Plasma glucose seldom gets high enough that glucose Tm is reached unless an individual has diabetes mellitus (see Chapter 16). Note that the kidney does not control blood glucose levels; it simply filters and reabsorbs all it can. The pancreas, via insulin release, controls blood glucose.
unit of time. For glucose, at a certain filtered load (GFR × plasma concentration), all carriers become occupied. Any glucose filtered beyond that load is not reabsorbed, but is instead excreted in the urine. The Tm for glucose is approximately 375 mg/min of filtered glucose. The concentration of glucose which results in this filtered load, given a GFR of 125 mL/min, is 3.0 mg/mL of plasma because glucose concentration clinically is frequently expressed as per 100 mL of blood, or, 300 mg/dL. However, glucose begins to appear in the urine even before this plasma level is reached, because each nephron has a slightly different Tm and the carrier transport rate may accelerate at the highest glucose concentrations. Plasma glucose seldom gets high enough that glucose Tm is reached unless an individual has diabetes mellitus (see Chapter 16). Note that the kidney does not control blood glucose levels; it simply filters and reabsorbs all it can. The pancreas, via insulin release, controls blood glucose.
In the kidney, glucose reabsorption is coupled with the reabsorption of sodium ions from the urine filtrate into the tubular cells. At some point this movement is driven by the splitting of adenosine triphosphate (ATP) by the sodium-potassium ATPase. It is a process that requires energy. It is this secondary use of energy that makes glucose transport an active (energy-requiring) process.
Reabsorption of Sodium
Sodium reabsorption occurs throughout the tubule by a combination of simple diffusion and active transport. Approximately 65% of sodium reabsorption occurs across the proximal tubule and 25% across the loop of Henle. Therefore, only approximately 10% of the filtered sodium remains in the tubule by the time the filtrate reaches the distal convoluted tubule. The final concentration of sodium in the urine is usually less than 1% of the total amount filtered at the glomerulus.
Unlike glucose, plasma sodium concentration is regulated by the kidney. Although sodium is freely filtered and 98% to 99% is normally reabsorbed, the final 1% to 2% of its reabsorption can vary. Plasma sodium concentration is 145 mmol/L, and the amount of filtered sodium is approximately 18 mmol/min (supposing a GFR of approximately 180 L/day). This amounts to approximately 1500 g of sodium filtered each day. Even if only 2% of this amount—30 g/day—is excreted, it is a considerable amount. This final 1% to 2% is controlled by the presence or absence of the hormone aldosterone.
Transport of sodium out of the nephron and back into the capillaries may either be coupled in the same direction to the reabsorption of another substance (cotransport), or it may be coupled in the opposite direction with another substance (counter-transport). Substances cotransported with sodium include glucose, amino acids, and chloride. Hydrogen ion (H+) is countertransported and thus secreted into the urine when a sodium ion is reabsorbed.
Reabsorption of Chloride
Chloride reabsorption can be active or passive and is nearly always coupled to sodium transport. It is affected by the electrical gradient across the tubule. Like sodium, most chloride reabsorption (65%) occurs across the proximal tubule, less across the loop of Henle (25%), and the rest (10%) between the distal convoluted tubule and the collecting-duct system.
Reabsorption of Potassium
Most potassium in the body is present intracellularly. Therefore, although plasma potassium is freely filtered across the glomerulus, its concentration in the Bowman capsule is low. Most potassium that is filtered is reabsorbed: 50% across the proximal tubule, 40% in the thick ascending limb, and the remaining 10% in the final part of the nephron, the medullary collecting duct. Most potassium reabsorption occurs by passive diffusion.
Potassium is also secreted into the tubule by active transport across the cells of the proximal tubule, the descending limb of the loop of Henle, and the collecting ducts. The amount of secreted potassium is variable and depends on the amount of potassium ingested in the diet. An individual on a high-potassium diet filters, reabsorbs, and secretes potassium. An individual on a low-potassium diet only filters and reabsorbs, but does not secrete, potassium. Potassium secretion by the collecting ducts is stimulated by the hormone aldosterone released from the adrenal cortex.
Reabsorption of the Amino Acids
Amino acids filtered at the glomerulus are actively reabsorbed in the proximal tubule. All reabsorption of amino acids is carrier-mediated. The Tm for the carriers is well above the amounts of amino acids normally filtered, so none are normally present in the urine.
Reabsorption of Plasma Proteins
Very few plasma proteins are filtered across the glomerulus. Those that are filtered are actively reabsorbed across the proximal tubule. Because the GFR is so high, the filtration of even a few molecules of plasma protein, such as albumin, would result in a significant daily loss of protein if reabsorption did not occur.
The few proteins filtered at the glomerulus are not reabsorbed. They are degraded by tubular cells and excreted in the urine. Examples of these proteins include the protein hormones, such as growth hormone and luteinizing hormone, both of which are secreted from the anterior pituitary.
Reabsorption of Urea
Urea is produced in the liver as an end-product of protein metabolism. It is freely filtered at the glomerulus. Because urea is highly permeable across most
(but not all) of the nephron, it diffuses back into the peritubular capillaries. It follows water as water is reabsorbed from the urine filtrate moving through the nephron. By the end of the proximal tubule, approximately 50% of the filtered urea has been reabsorbed. From the end of the proximal tubule to the medullary collecting ducts, the proximal tubule is impermeable to urea. Along this route, some portions of the tubule begin to secrete urea into the filtrate. Thus, at the point the filtrate reaches the medullary collecting ducts, urea concentration has again reached what it was in the original glomerular filtrate. At the medullary collecting ducts, urea once more becomes permeable and again follows water reabsorption out of the tubule. As the filtrate leaves the kidney, approximately 40% of the original filtered urea remains and is excreted.
(but not all) of the nephron, it diffuses back into the peritubular capillaries. It follows water as water is reabsorbed from the urine filtrate moving through the nephron. By the end of the proximal tubule, approximately 50% of the filtered urea has been reabsorbed. From the end of the proximal tubule to the medullary collecting ducts, the proximal tubule is impermeable to urea. Along this route, some portions of the tubule begin to secrete urea into the filtrate. Thus, at the point the filtrate reaches the medullary collecting ducts, urea concentration has again reached what it was in the original glomerular filtrate. At the medullary collecting ducts, urea once more becomes permeable and again follows water reabsorption out of the tubule. As the filtrate leaves the kidney, approximately 40% of the original filtered urea remains and is excreted.
Note that urea reabsorption depends on water reabsorption. If water reabsorption is low, more urea will be excreted, and vice versa.
ACID-BASE HANDLING
The kidney plays a pivotal role in maintaining acid-base balance. Most metabolic processes in the body produce acid (see Chapter 19). These processes include oxidative phosphorylation, which produces the volatile acid carbon dioxide, and the metabolism of proteins, which produce nonvolatile acids such as sulfuric and phosphoric acids. Although the lungs normally excrete all carbon dioxide produced by oxidation, the kidney is the only organ capable of eliminating nonvolatile acids. More importantly, the kidneys have the essential job of reabsorbing large quantities of the base bicarbonate, which is freely filtered at the glomerulus. Without this function, fatally low blood pH would occur. The kidneys assist in eliminating acid produced by cell metabolism in individuals who have lung disease by increasing the secretion and excretion of acid and by reabsorbing increased amount of base.
Reabsorption of Bicarbonate
Reabsorption of bicarbonate is an active process that occurs primarily in the proximal tubule (and to a lesser extent in the collecting ducts). As shown in Figure 18-6, reabsorption occurs when a molecule of water breaks down in the proximal tubular cell into an H+ and a hydroxyl molecule (OH−). The H+ is actively secreted into the lumen of the tubule and joins with a bicarbonate molecule that has been filtered at the glomerulus. Hydrogen plus bicarbonate results in carbonic acid (H2CO3), which, in the presence of the enzyme carbonic anhydrase, breaks down to carbon dioxide and water. These diffuse back into the proximal tubular cell to be used again as this cycle repeats.
By this process, the filtered bicarbonate is saved from being excreted in the urine. The reaction of hydrogen with bicarbonate is reversible, as shown in the following equation:

The OH− produced in the proximal tubule cell joins with an intracellular carbon dioxide molecule. In the presence of the enzyme carbonic anhydrase, it too proceeds to a bicarbonate ion. This bicarbonate also returns into the peritubular capillary as shown in Figure 18-6. The enzyme carbonic anhydrase is readily available.
Secretion and Excretion of Acid
The above reactions only serve to reabsorb filtered bicarbonate. They do not eliminate acid. The kidney does actively secrete and excrete H+ in the urine as well, which allows it to rid the blood of metabolically produced nonvolatile acids. As shown in Figure 18-7, H+ excretion occurs after most of the filtered bicarbonate has been reabsorbed. In this case, the H+ produced in the proximal tubule cell from the breakdown of water moves into the lumen of the tubule and combines with filtered phosphate ions (or to a lesser extent, sulfate ions) and is then excreted in the urine.
The effect of excreting hydrogen bound to phosphate is not only the loss of acid in the urine but a net gain of bicarbonate. This net gain occurs because a bicarbonate ion is still produced in the proximal tubule when carbon dioxide joins with OH−. This bicarbonate is returned to the plasma.
A second mechanism by which the kidney excretes acid is by active secretion of ammonium ion (NH4+) into the tubular fluid (Fig. 18-8). Ammonium ion is produced in the proximal tubular cell as a result of the metabolism of glutamine. Glutamine enters the cell from the peritubular capillary and from the tubular lumen, after being filtered across the glomerulus. Once in the tubule, ammonium ion cannot return into the proximal tubular cells; therefore, it is excreted in the urine. The bicarbonate produced from glutamine metabolism diffuses back into the peritubular capillary, thereby returning base to the blood. Finally, a small amount of H+ is excreted free in the urine, causing the urine to normally have an acidic pH.
Secretion of Bicarbonate
Under conditions of alkalosis (excess base), the kidney can secrete bicarbonate, thus ridding the plasma of base and returning the pH toward normal. Secretion
of bicarbonate is an active process occurring in the cortical collecting duct. However, even under conditions of alkalosis, bicarbonate reabsorption in the proximal tubule is ongoing and essential. Loss of all filtered bicarbonate would be fatal.
of bicarbonate is an active process occurring in the cortical collecting duct. However, even under conditions of alkalosis, bicarbonate reabsorption in the proximal tubule is ongoing and essential. Loss of all filtered bicarbonate would be fatal.
RENAL CONCENTRATING MECHANISM: THE COUNTERCURRENT SYSTEM
To survive periods without water, animals, including humans, must excrete a concentrated (hypertonic) urine. They must eliminate waste products, including urea, without losing much water in the process. In contrast, under conditions of water excess, animals must excrete large amounts of water in a dilute (hypotonic) urine. The kidney has adapted to handle day-to-day variations in water consumption by developing the countercurrent multiplier system. For this system to work, the hormone antidiuretic hormone (ADH), also called vasopressin, is required.
The countercurrent multiplier system exists in the loop of Henle, a long, curving portion of the nephron located between the proximal and distal tubules. The multiplier system has five basic steps and depends on active transport of
sodium (and chloride) out of the ascending part of the loop. It also depends on impermeability of this part of the loop to water, which keeps water from following sodium out. Finally, this system relies on the permeability of collecting ducts to water. The five steps are outlined in the following section and are shown graphically in Figure 18-9.
sodium (and chloride) out of the ascending part of the loop. It also depends on impermeability of this part of the loop to water, which keeps water from following sodium out. Finally, this system relies on the permeability of collecting ducts to water. The five steps are outlined in the following section and are shown graphically in Figure 18-9.
![]() FIGURE 18-9 Formation of a concentrated urine in the presence of ADH. With ADH, water diffuses out of the collecting duct into the concentrated interstitium. |
Steps of the Countercurrent Multiplier System
When sodium is transported out of the ascending limb, the interstitial fluid surrounding the loop of Henle becomes concentrated.
Because water is impermeable across the ascending limb, water cannot follow sodium out of the ascending limb. Thus, the remaining filtrate becomes progressively diluted.
Water is permeable across the descending limb of the loop. Water leaves this section and flows down its concentration gradient into the surrounding interstitial space. This concentrates the descending limb fluid. As the fluid loops into the ascending limb, it is progressively diluted as sodium is pumped out.
The net and key result is the concentration of the interstitial fluid surrounding the loop of Henle. Concentration is highest surrounding the bottom of the loop, becoming more dilute as the ascending limb is followed up.
At the top of the ascending limb, tubular fluid is isotonic (equal in concentration to the plasma) or even hypotonic (more dilute compared with plasma).
Result of the Countercurrent Multiplier System
The goal of the countercurrrent system is to concentrate the interstitial fluid surrounding the loop of Henle (as described in step 4). This is vital because the final filtrate passes down the collecting ducts through this fluid. Permeability of the collecting ducts to water is variable. If permeability to water is high (as shown in Fig. 18-9
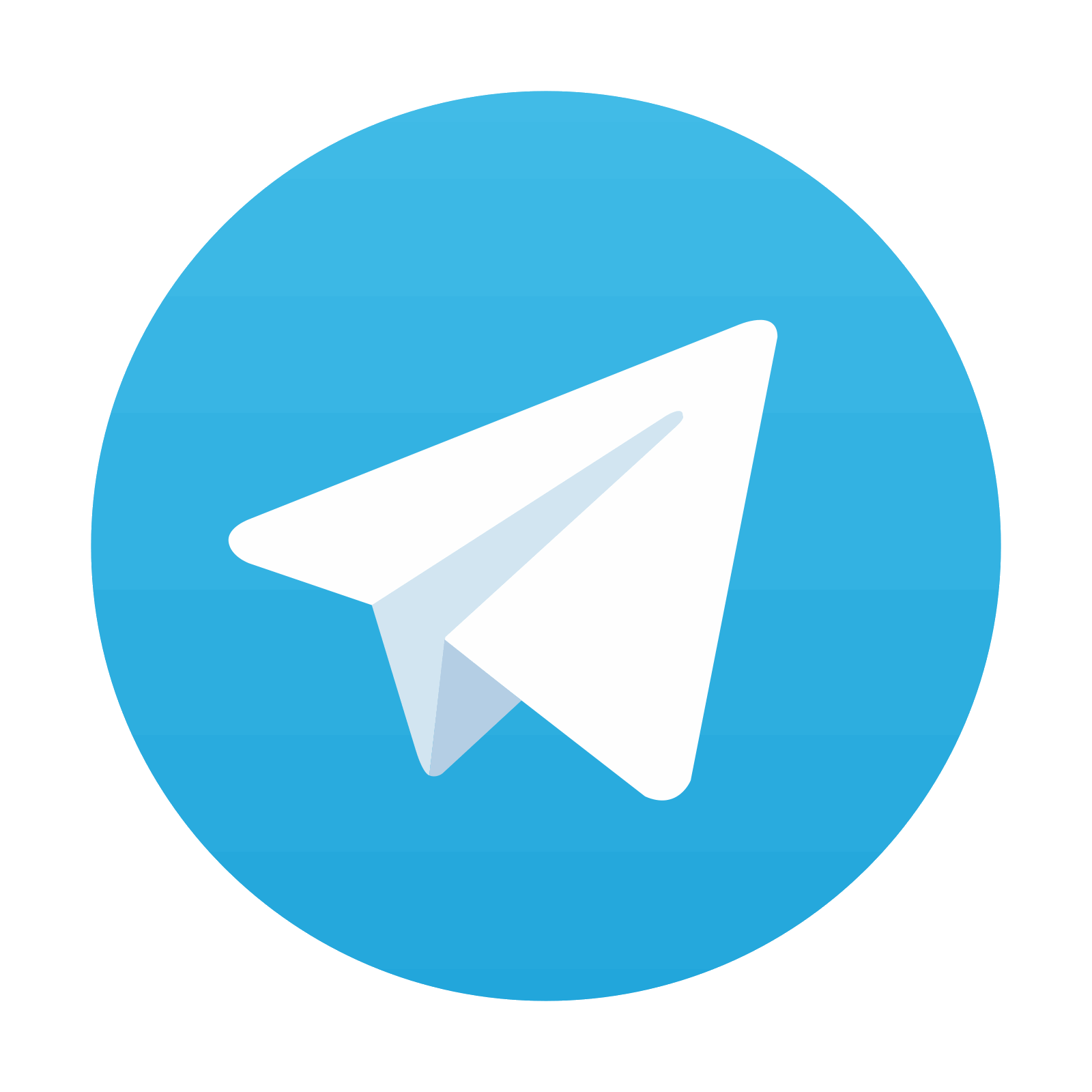
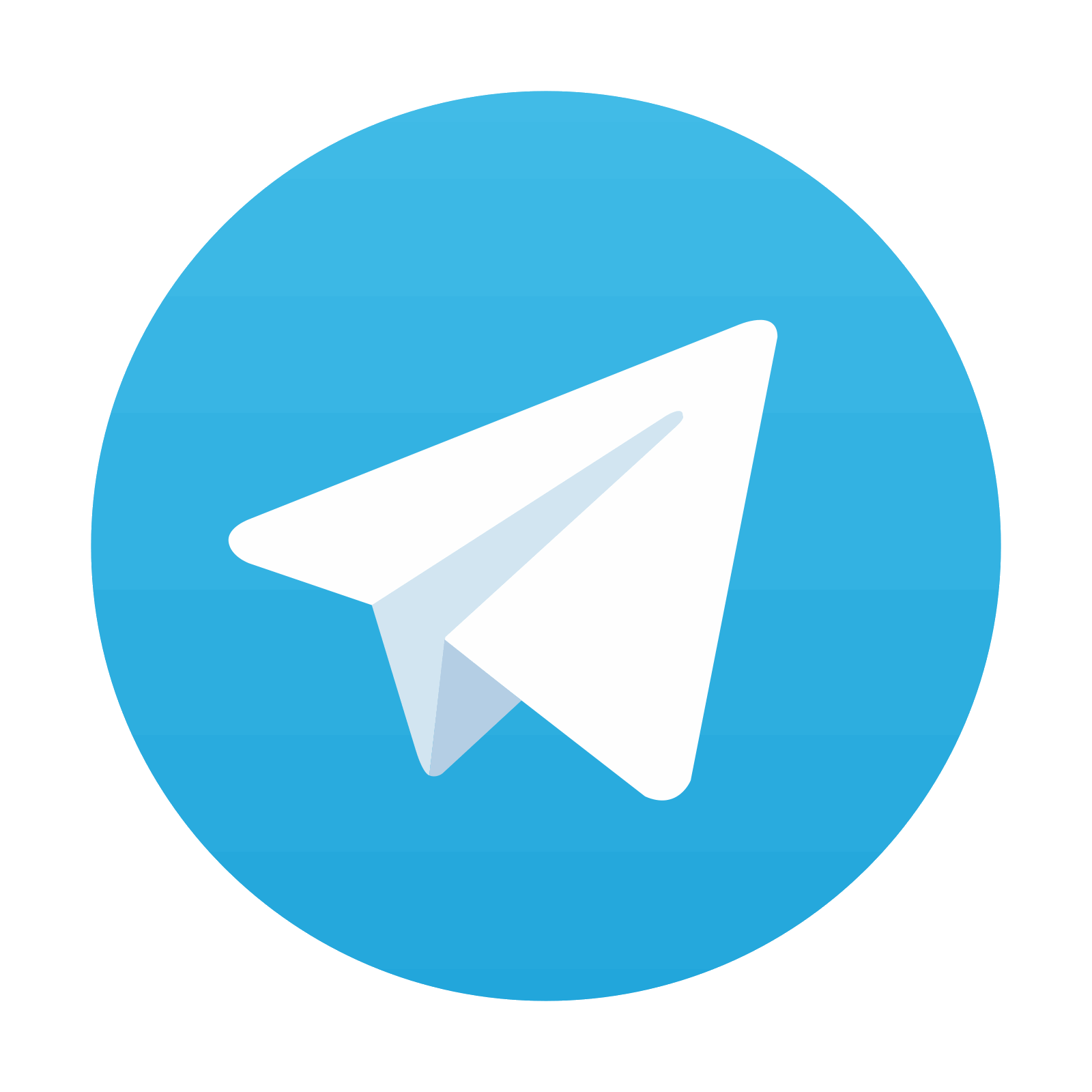
Stay updated, free articles. Join our Telegram channel
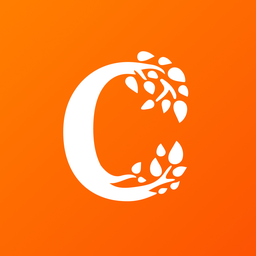
Full access? Get Clinical Tree
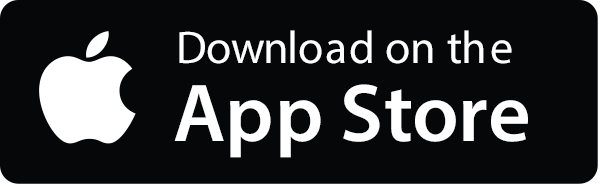
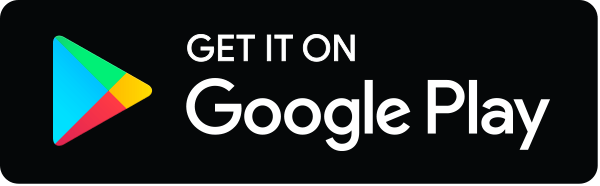
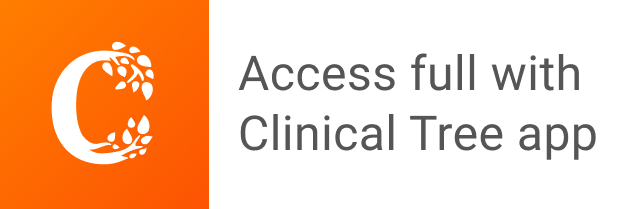