Fig. 38.1
(a and b) The da Vinci Si HD surgical system: surgeon console and patient cart (© 2009 Intuitive Surgical, Inc., with permission)
Other master–slave systems currently being developed include:
The Amadeus by Titan Medical Inc. (Canada) which is a robotic surgical system with multi-articulating arms and single-site and multi-port platform capabilities with infrared and ultrasound imaging and enhanced 3D visualization. Additional features include force feedback and advanced communication technology for patient data, telesurgery, and training [5, 7]. It has been indicated that clinical testing will be initiated in the next 2 years [8]. Titan Medical is also developing a single incision robotic surgical platform with 25 mm access, 3D visualization, and interactive micro-instruments (Fig. 38.2). Projected release of the platform is 2015 [7].


Fig. 38.2
Titan single incision surgery platform, reprinted with permission
ALF-X (SOFAR S.p.A, Italy) (Fig. 38.3a, b) is a new surgical system undergoing testing in Europe. It enhances surgical dexterity with a realistic tactile-sensing capability due to a patented approach which measures tip/tissue forces, with a sensitivity of 35 g and advanced eye tracking to control view [9]. The robotic system contains four independent arms with adaptable surgical instruments for clinical use in gynecology, urology, and thoracic surgery. With animal trials complete, and indicating significantly reduced procedural time for cholecystectomy compared to the conventional telesurgical system (average of 31.75 min versus 91 min) [10], the ALF-X has now received CE mark and is set to reach market soon [11].


Fig. 38.3
(a and b) The ALF-X Telelap robot, reprinted with permission
The DLR MIRO system (the Institute for Robotics and Mechatronics, Germany) incorporates haptic feedback through force and tactile feedback or optical tracking [5]. The MiroSurge (Fig. 38.4) is compact and lightweight, consisting of three of the MIRO robotic arms; generally two are used for surgical tools and one for visualization. Each arm has seven torque-controlled joints for flexible movement designed to mimic the human arm. Presently this platform is used for research purposes. Technical advances may include compensation for heartbeat and motion to allow cardiac surgery without stopping the heart [12].


Fig. 38.4
Three DLR MIROs in a setup for minimally invasive surgery (from DLR Institute of Robotics and Mechatronics with permission)
Similarly, developed but not yet available commercially is a remote-operated robotic surgical system named “Sofie”: Surgeon’s Operating Force-feedback Interface Eindhoven developed by the University of Technology in Eindhoven. The 4D manipulators (surgical arms and a camera) are mounted on the operating table and include a distinctive tactile force feedback system of counter pressure through the joystick controllers [5, 13]. Although this prototype has not yet been released to market, it is anticipated that an advantage of this compact system will be a reduced cost to larger current models [13].
The RAVEN II (the University of Washington and UC Santa Cruz) (Fig. 38.5) consists of two cable-based, articulated aluminum dual arms with 7 DOF, with exterior motors, reducing the size and weight. The robotic prototype is capable of teleoperation and has been released to research labs, with initial application in cardiac surgery, aspiring to include beating heart procedures, with motion compensation and 3D ultrasound imaging capabilities [14, 15].


Fig. 38.5
The Raven II, The University of Washington (from: Jacob Rosen, Mika Sinanan, and Blake Hannaford, Objective Assessment of Surgical Skills, Chapter 25 in Surgical Robotics, Systems, Applications, and Visions, Jacob Rosen, Blake Hannaford, Richard M. Satava (Editors), 1 ed. Springer 2011; Zhi Li, Daniel Glozman, Dejan Milutinovic, and Jacob Rosen, Maximizing Dexterous Workspace and Optimal Port Placement of a Multi-Arm Surgical Robot, ICRA 2011, Shanghai, China, May 2011; H. Hawkeye King, Lei Cheng, Philip Roan, Diana Friedman, Sina Nia Kosari, Ji Ma, Daniel Glozman Jacob Rosen, Blake Hannaford, Raven II™: Open Platform for Surgical Robotics Research, The Hamlyn Symposium on Medical Robotics, July 1–2 2012, London, UK; H. Hawkeye King, Blake Hannaford, Ka-Wai Kwok, Guang-Zhong Yang, Paul Griffiths3, Allison Okamura, Ildar Farkhatdinov, Jee-Hwan Ryu, Ganesh Sankaranarayanan, Venkata Arikatla, Suvranu De, Kotaro Tadano, Kenji Kawashima, Angelika Peer, Thomas Schuß, Martin Buss, Levi Miller, Daniel Glozman, Jacob Rosen, Thomas Low, Plugfest 2009: Global Interoperability in Telerobotics and Telemedicine, IEEE International Conference on Robotics and Automation, ICRA May 2010, Alaska, USA with permission)
The NeuroArm (developed at the University of Calgary, AB in collaboration with MacDonald, Dettwiler and Associates Ltd) was one of the first MRI-compatible surgical robotic systems. The master–slave design of this system does not allow freedom of movement by the operating surgeon, but force feedback and real-time imaging combined with preoperative diagnostic images do provide effective tools for reference during the procedure [16]. Used in clinical trial for dissection during microsurgery, successful results were reported with the 34 cases, with one adverse event due to uncontrolled motion of the arm and one conversion to the standard procedure due to reduced access and trouble with positioning during the procedure (Sutherland et al. [17]). The NeuroArm technology was acquired by IMRIS Inc. (NASDAQ: IMRS; TSX: IM) in 2010, after which the SYMBIS neurosurgical robot system was released in 2012 (by IMRIS), pending FDA approval [18].
The Robin Heart 2 robot (developed by the Foundation for Cardiac Surgery Development, Zabrze, Poland) consists of two or more arms for tools and a camera, allowing the surgeon to essentially perform three roles (of two surgeons and an endoscopic assistant) [19]. Future development includes computer simulation and operative planning with application in cardiac surgery for valve repair and replacement, laser revascularization, and possible future utilization in drug delivery and robotic-assisted artificial organ surgery [20].
Continued research and development efforts into the master–slave surgical robotic system and the application of Laparo-Endoscopic Single-Site Surgery (LESS) and Natural Orifice Translumenal Endoscopic Surgery (NOTES) to further reduce surgical invasiveness, and decrease port and trocar access, have brought forth new robotic prototypes. Ideally, robotic platforms for LESS should have the desired instrument maneuverability, dexterity, and freedom of movement and restored triangulation without instrument clashing for precise movement [21]. Designs to current systems have been improved to include pre-bent curved, flexible, and articulating arms to increase maneuverability. Clinical application of robotic-assisted NOTES has been demonstrated in preclinical and clinical study using various approaches. Transgastric, transvesical, and transcolonic approaches have been used in abdominal surgery, colonoscopy, and endoscopy [22].
An agile transluminal endoscopic robot (Master And Slave Transluminal Endoscopic Robot, MASTER) (Fig. 38.6a, b) was developed by Singapore’s Nanyang Technological University and National University Hospital to overcome some of the limitations of standard endoscopic devices, including the lack of triangulation [23]. The controller of this system attaches to the wrist and fingers of the operating surgeon, allowing for 9 DOF. Animal trials using the MASTER for endoscopic submucosal dissection (ESD) with NOTES have been conducted with promising results [24], and early results from human trials showed evidence of a shortened procedure time [25]. This system is anticipated to be released to market in the near future.


Fig. 38.6
(a and b) The robotic slave manipulator with two steerable articulating arms and controller (MASTER) (From Felice Cosentino, Emanuele Tumino, Giovanni Rubis Passoni, Antonella Rigante, Roberta Barbera, Antonella Tauro and Philipp Emanuel Cosentino (2011). Robotic Colonoscopy, Colonoscopy, Paul Miskovitz (Ed.), ISBN: 978-953-307-568-6, InTech with permission)
A computer-assisted robotic technology system, the NeoGuide (NeoGuide Systems Inc., San Jose, CA, USA), has been developed and approved for application during colonoscopy [26]. The system incorporates a tip sensor that continually records the steering commands and an external sensor that records the insertion depth to guide the scope along the natural shape of the colon [27]. A compact endoscopic robot ViKY (EndoControl Medical, France) has been developed, recently obtaining CE mark and FDA approval [28]. The system can be voice controlled or foot switch operated to robotically control the laparoscopic camera with an innovative instrument tracking system [29].
Advances in surgical robotic systems have generated the integration of preoperative and intraoperative imaging for procedural guidance and tracking of tools during surgical intervention while accounting for organ movement [30]. A body-global positioning system for navigation and organ tracking has been pioneered by Ukimura and Gill [30]. This ability to integrate preoperative imaging can also be beneficial in pre-planning, training, and mentoring. Many systems utilize the overlaid images obtained through computerized tomography (CT), magnetic resonance imaging (MRI), or ultrasound for procedural guidance. A system for the 3D imaging of tissue in real time using fluorescence imaging has been developed by Intuitive Surgical Inc. for the da Vinci system and is currently in trial, imaging renal cortical tumors to determine the optimal dose of ICG fluorescence for visualization using the SPY scope [31].
Direct Image-Guided Robots
Robotic systems designed for intervention under image guidance are an exciting development, providing targeted therapy with clinical application in oncology, urology, gynecology, general surgery, neurology, and cardiology.
The Cyberknife system (Accuray, Inc., Sunnyvale, CA) (Fig. 38.7) was the first image-guided robotic technology for noninvasive radiation procedures, receiving FDA approval in October of 2001. The system consists of a large robotic arm that can quickly and accurately deliver targeted radiation with converged beams from multiple angles, reducing radiation exposure to surrounding tissue [32]. A real-time image guidance system and respiratory tracking system identifies the position of the patient, making continual adjustments for movement. Established use in brain tumors as well as lesions of the spine, lung, pancreas, liver, and prostate has been reported [33].


Fig. 38.7
CyberKnife system, Accuray Inc., reprinted with permission
The commercially available, FDA-approved, miniature robot, Spine Assist (Mazor Robotics Ltd, Israel) (Fig. 38.8), is a bone-mounted hexapod robot which assists in navigation and guidance for thoracic and lumbar pedicle screw drilling and implantation, vertebral biopsies, and kypho- and vertebroplasties [34]. Future possible developments may include application in craniocervical surgery and cervical and lumbar total disc replacement [34]. Based on experience with the SpineAssist, Mazor Robotics has introduced the Renaissance platform (Mazor Robotics Ltd) which creates a 3D preoperative blueprint of the procedure, synchronizing with the instrumentation to provide an implant procedure within 1 mm of accuracy [35]. The Renaissance platform received CE mark in 2011 and US FDA marketing clearance in 2012 to be used for application in brain and spinal surgery [35].


Fig. 38.8
The SpineAssist (Mazor Robotics Ltd, Israel) (From Stüer C, Ringel F, Stoffel M, Reinke A, Behr M, Meyer B. Robotic technology in spine surgery: current applications and future developments. Acta Neurochir Suppl. 2011;109:241–5 with permission)
The RIO (Robotic Arm Interactive Orthopedic System) (MAKO Surgical Corp., USA) received FDA clearance in 2008, for knee joint resurfacing during partial knee and total hip replacement procedures, providing patient-specific pre-planning [36]. The application uses pre-procedure imaging data to create a cutting guide, with integrated digital tracking of the procedure and constant monitoring utilizing visual, tactile, and auditory feedback. The robotic system does not require bone fixation [36], and the feedback resistance system restricts the surgeons’ movement to within the planned cutting area [36].
While magnetic resonance (MR)-guided percutaneous interventions, such as biopsies and ablation, have been clinically demonstrated with open-bore systems [37, 38], the closed-bore MR imaging (MRI) scanners provide superior resolution, but offer limited access during imaging and therefore limited feasibility for intervention other than robotic [39]. Innomotion (Innomedic, Herxheim & FZK Karlsruhe Germany & TH Gelsenkir), a CT and MR-compatible robotic instrument-guiding system, was developed to provide precise and reproducible instrument positioning inside the magnet [39]. The Innomotion robotic arm is mounted on specifically designed bed rails, with the transducer attached to the robotic arm guided by the operating surgeon, utilizing MR images and Innomotion software [40].
An MRI-compatible, image-guided, pneumatic, remotely operated robot, the MrBot, has been designed to employ high-precision, image-guided access of the prostate gland, accommodating various needle drivers for different percutaneous interventions such as biopsy, thermal ablations, and brachytherapy [41]. Early results show good accuracy with the image-guided needle and seed placement during testing in a 3 T MRI scanner [42], but no information on commercial availability can be found.
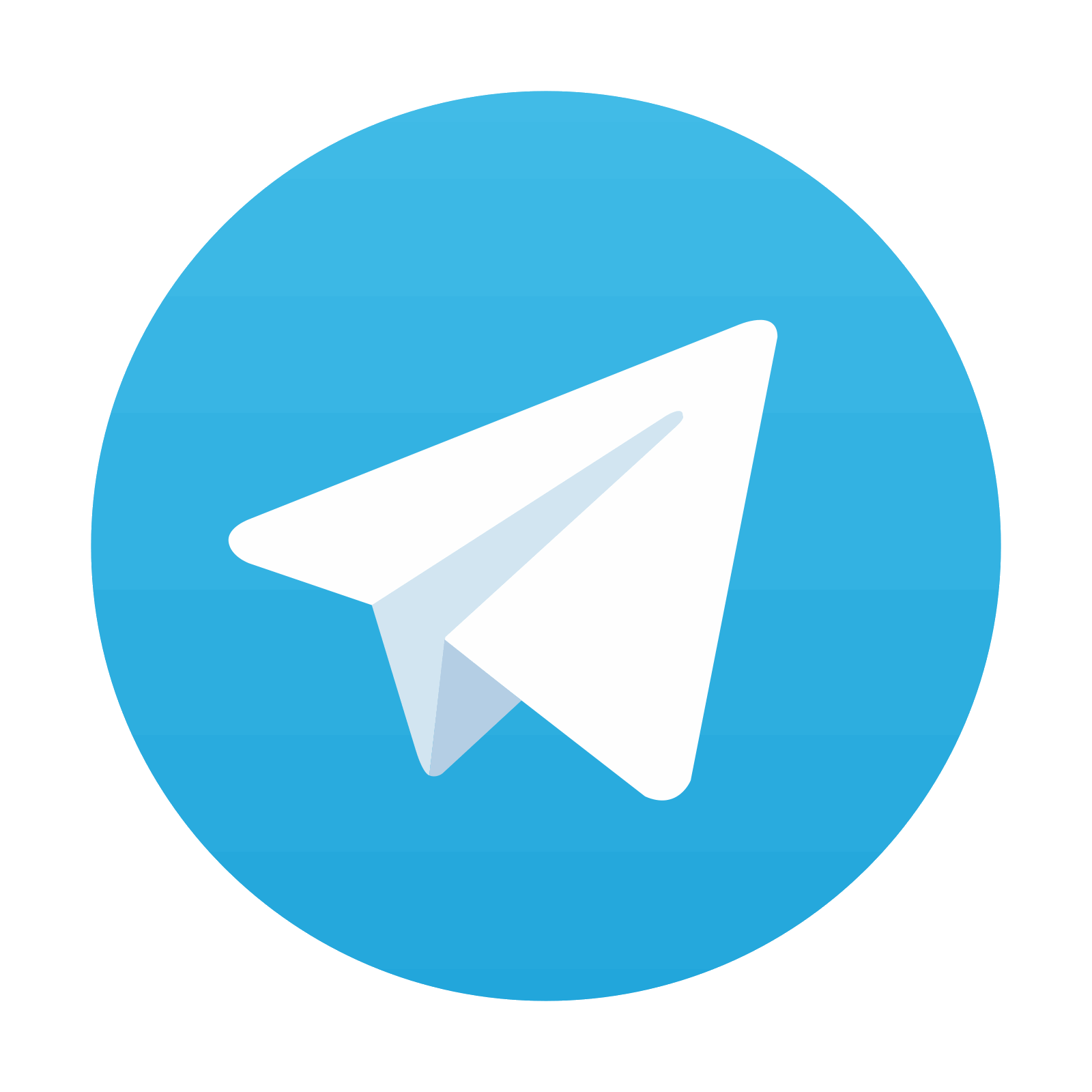
Stay updated, free articles. Join our Telegram channel
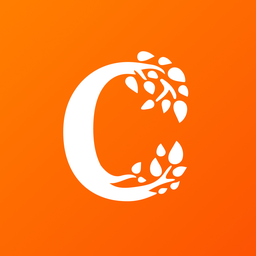
Full access? Get Clinical Tree
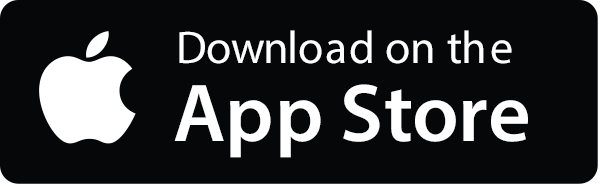
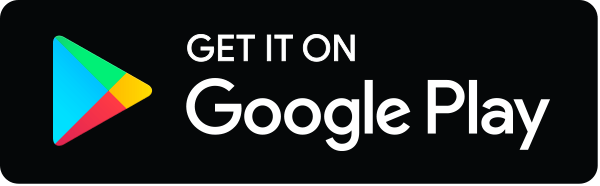