Fig. 6.1
The tumor microenvironment. Here we show the variety of host-derived stromal cells that are involved in mediating tumor progression. Tumor cells secrete factors that remodel the ECM and alter the behavior of stromal cells to often aid tumor development. These host-derived tumor-associated cells then further facilitate tumor growth/invasion by secreting additional factors that promote tumor proliferation, invasion, angiogenesis, remodeling of the ECM, and recruitment of tumor-promoting immune cells
The ECM
The ECM is comprised of many types of proteins, which are usually large in size, complex, and highly conserved between species [11]. These include laminins, collagens, fibronectin, elastin, and tenascins. The ECM was once viewed as merely a stable supporting structure that maintains tissue morphology, but recent studies have demonstrated the dynamic nature of the ECM, which undergoes extensive remodeling that is tightly controlled, especially during normal tissue homeostasis [12]. Aberrant ECM dynamics, such as increases in ECM deposition, altered cross-linking of ECM molecules, and changes in stiffness, leads to abnormal behaviors of the cells nearby. This results in altered integrin signaling as well as altered growth factor signaling due to changes in the presentation of growth factors bound to the ECM [11]. Indeed, abnormal ECM is often a clinical precursor of diseased states including fibrosis and cancer [13].
Carcinoma-Associated Fibroblasts
Carcinoma-associated fibroblasts (CAFs) can be derived from multiple cell types including fibroblasts in the immediate tumor environment, bone marrow-derived cells, endothelial cells (through an endothelial-to-mesenchymal transition, or EndMT), and perhaps also from the cancer cells through epithelial-to-mesenchymal transition (EMT) [6]. Compared to normal fibroblasts, CAFs typically promote tumor growth and angiogenesis and enhance the metastatic dissemination of cancer cells [5, 6], although these tumor-supportive effects may be tumor-type dependent, as recent research suggest that CAFs inhibit pancreatic cancer progression in certain contexts [14, 15]. CAFs may also travel in the circulation as fibrocytes and enhance tumor proliferation and fibrosis (i.e., chronic inflammation) once recruited to the tumor site [16]. Certain subtypes of fibrocytes may also mediate immunosuppression, while maintaining phenotypic and functional hallmarks of a traditional fibrocyte [17], and prepare secondary organ sites for metastasizing cancer cells through recruitment of monocytes [18].
Bone Marrow-Derived Cells
The bone marrow is a source for highly plastic cells that are mobilized into circulation and then migrate toward tumors. Bone marrow-derived cells (BMDCs) are recruited during the beginning stages of tumor development [19]. It is suggested that as they are incorporated into the TME, they differentiate into various different cell types including pericytes, endothelial cells, fibroblasts, and monocytes, which contribute to tumor growth and metastasis through diverse mechanisms [8]. Interestingly, BMDCs recruited by cancer cells may not necessarily “home” toward the primary tumor; instead, they may colonize specific areas in metastatic sites, in preparation for incoming metastasized cancer cells, creating what are known as “pre-metastatic niches ” [20, 21].
Immune Cells
Immune cells from both the innate (e.g., macrophages and neutrophils) and adaptive immune system (e.g., T and B cells) are important defenders against bacteria, viruses, and cancer cells. As expected, immune therapies for cancer have been focused on, for example, harnessing the cytotoxic abilities of T cells (cytotoxic T lymphocytes or CTL) against tumor cells [22]. Emerging evidence has shown that the TME can co-opt and subvert these immune cells to promote tumor progression, by altering their polarization/activation status. In general, immune cells with “1” in their polarization nomenclature indicate an antitumor phenotype, whereas “2” denotes pro-tumor properties. For example, T-helper cells, which regulate the activation and proliferation of CTLs, can be Th1 or Th2. Th1-polarized cells can elicit direct cytotoxic effects against tumor cells and are responsible for activation and perseverance of the CTL population [23]. Th2-polarized cells on the other hand activate a humoral response (i.e., B-cell activation as opposed to CTL activation) and suppress Th1 responses [7, 23]. For macrophages, there are at least two types of polarization: “M1” and “M2.” M1s secrete inflammatory cytokines that activate Th1-adaptive immune response, which in turn kills tumor cells [24]. Conversely, tumor-associated macrophages (TAMs) that have infiltrated tumors are typically M2 polarized [24]. M2s secrete immunosuppressive cytokines and stimulate a Th2 response, thus inhibiting the Th1 response while promoting tumor growth. M2s also secrete various proteases and angiogenic factors, which promote invasion and metastasis of the tumor cells [25]. Similar to macrophages, it has recently been demonstrated that neutrophils are also “N1” and “N2” polarized [26]. N1s have much higher cytotoxic activities against tumor cells and secrete more immune-activating cytokines/chemokines, and it was shown that TGFβ within the TME recruits the pro-tumor N2 phenotype, which in contrast to N1s contributes to immunosuppression and thus promotes tumor progression [26].
Vascular Cells
The involvement of vascular cells (endothelial cells and pericytes) in tumor progression is well-studied [27]. Tumor growth requires oxygen and nutrients, and the ability of tumors to undergo an angiogenic switch (i.e., become vascularized) is a crucial step during tumor progression. This requires both co-option of nearby vessels as well as new endothelial cells, in a process known as sprouting [28, 29]. Recently, it was shown that genetic depletion of Apelin (Apln) specifically in the vasculature diminished sprouting and reduced tumor growth [30]. Furthermore, tumor vessels are needed to remove waste products generated during tumor metabolism as well as provide a route for metastasizing cancer cells throughout the body [9]. Interestingly, tumor endothelial cells (TECs) have very different biology compared to normal endothelial cells; for example, TECs have a unique gene expression signature, and vessels formed by TECs have excessive branching and increased leakiness [31, 32]. In addition, aberrant cross talk between TECs and immune cells such as leukocytes, T cells, and macrophages provides the tumor with both protection against immune surveillance while promoting a pro-inflammatory environment, which further fuels tumor progression [9].
Adipocytes and Cancer: An Introduction
Adipocytes have been mostly overlooked or considered innocent bystanders in the TME. This is despite the fact that adipocytes are abundant in multiple cancer types including breast, ovarian, prostate, and liver [33]. Additionally, obesity , a medical condition characterized by expansion and hypertrophy of adipocytes, is a risk factor for certain cancers [34, 35]. The prevalence of obesity has increased drastically worldwide as a result of increased affluence of the general population, as well as a shift in dietary habits. It is estimated that up to 20% of all cancer cases count obesity as a dominant causative factor [36, 37], which impacts not only cancer incidence but also treatment outcomes [38]. The relationship between obesity and cancer is complex, as an increase in body mass index (BMI) may impact cancer progression either positively or negatively depending on the type of cancer (see [33, 38]). It is known that BMI does not necessarily reflect adiposity or reflect distribution of adipose tissues accurately [39], and the latter is a risk factor as well as prognostic indicator for colorectal cancer [40], underlining the importance of adipose tissue location as well as overall adiposity in mediating cancer progression.
In kidney cancer (renal cell), obesity is a strong risk factor for cancer incidence; paradoxically, in patients already diagnosed, obesity is linked to extended survival [41]. The cause of this phenomenon is still under debate and may be attributed to the “nutritional buffer” provided by the extra adipose tissue for cancer patients undergoing chemotherapy and/or radiation therapy; this decreases treatment-related toxicities and increases treatment efficacy [38, 42]. Irrespective of confounding and complex results from epidemiologic meta-analyses associating obesity and cancer, obesity is clearly a cancer risk factor and is associated with increased mortality for kidney, colon, liver, prostate, and breast cancers [36].
The importance of white adipose tissue (WAT, see the section “Types and Functions of Adipocytes” for different types of adipocytes that comprise adipose tissue) was questioned when studies using an A-Zip/F-1 “fatless” mouse demonstrated that these mice have accelerated tumor formation compared to wild-type mice [43, 44]. The authors concluded from these observations that adipokines, which are soluble factors secreted mainly by white adipocytes, are not important in mediating tumor progression. Instead, the authors argued, it is the inflammatory status that contributes to obesity-related cancer progression [44, 45]. However, the A-Zip/F-1 mice are severely diabetic, they have elevated systemic glucose/insulin/free fatty acids/triglycerides, and they have severe chronic inflammation as indicated by the highly elevated levels of inflammatory cytokines present in the serum. Surprisingly, despite being “fatless,” the A-Zip/F-1 mice have a much higher body weight as well as body length compared to their age-matched littermates [44]. These observations indicate that perhaps the results using the A-Zip/F-1 mice are not sufficient to conclude what role white adipose tissue plays in cancer progression, as any effects of adipocyte-related influence on tumor development may have been masked by the “cytokine storm” that characterizes this model. In addition, the adipokine adiponectin was significantly lower in the A-Zip/F-1 mice when compared to the wild-type mice. While this is not surprising as WAT is the predominant source of adiponectin, obese individuals were reported to have significantly lower circulating adiponectin levels [46], likely due to negative regulation by multiple adipose tissue-derived factors that are elevated during obesity (e.g., TNF-α, IL-6). Additionally, adiponectin levels in general are inversely correlated with cancer, suggesting that adiponectin may have tumor-inhibitory effects (discussed in details in the section “Adipokines and Cancer” of this chapter).
Studies from a few decades ago demonstrated that both spontaneous and induced mammary carcinomas are increased in Avy mice [47–50], which have ubiquitous expression of the appetite-stimulating agouti protein and thus have a much higher body weight [51]. Mice made obese by injection of gold thioglucose (GTG) also have a higher incidence rate for spontaneous mammary tumor development [52], although when the ovaries were removed from the obese mice, the cancer incidence was significantly lower than the control mice. This result suggested a confounding effect of sex hormones on obesity-related tumorigenesis [53]. Additionally, obese mice, induced by a high-fat diet, also had the highest rate of tumor growth when compared to lean mice [54]. Similar tumor-promoting effects of obesity were observed in various other cancers such as colorectal [55–57], liver [58, 59], prostate [60–63], and skin (both melanoma and non-melanoma, [64, 65]). These studies all suggest an important role for white adipose tissue and/or obesity in mediating cancer progression.
The Structure of Adipose Tissues
There are three main types of adipose tissues: (1) subcutaneous, which is below the skin and includes deep hypodermic depots; (2) mammary, which as the name suggests, is present in the breast; and (3) visceral, which surrounds the inner organs and is divided into omental, mesenteric, retroperitoneal, gonadal, perivascular, and pericardial depots [66]. In addition to these adipose depots, the bone marrow also contains a rich source of adipocytes, ranging from 15 to 60% of bone marrow volume in humans depending on age [67]. Adipose tissue was long thought of as mostly inert—providing insulation for the body against the cold, giving support and protection for vital organs, passively maintaining energy homeostasis by acting as a reservoir to store excess nutrients in the form of fat, and releasing the fat when required in the form of fatty acids. However, adipose tissue is rather a dynamic “organ” that actively secretes both paracrine and endocrine factors, which exerts an influence at both local and systemic levels; as such, dysfunction of the adipose tissue leads to metabolic syndromes such as hyperglycemia (increased blood glucose), dyslipidemia (increased circulating lipids), and insulin resistance [68]. Damaged or dysfunctional adipose tissues (e.g., adipocyte hypertrophy due to obesity) also release pro-inflammatory cytokines, which contribute to an inflammatory environment. The effects of WAT in cancer may not only be due to the systemic response of the body toward the obese condition (i.e., metabolic syndromes leading to the imbalance of hormones) but also due to a local response (e.g., increase in adipose-derived factors surrounding the tumor which alter the TME).
Adipose tissue is comprised of a heterogeneous population of cells, the predominant cell type being adipocytes. The other component, the stromal vascular fraction (SVF) , contains endothelial cells, lymphocytes, resident monocytes, macrophages, fibroblasts, and other precursor/progenitor cells [69]. Adipose SVF, in particular adipose progenitor cells, has been shown to promote tumor progression. For example, it was demonstrated that SVF cells from WAT is actively recruited by cancer cells to the primary tumor site, they are incorporated into specific niches created by various stromal cells (e.g., the vasculature), and they promote tumor growth [70]. In diet-induced obese mice engrafted with tumors, the number of circulating adipose-derived SVF cells increased dramatically, which were then incorporated into the developing primary tumor. This recruitment contributed to the stabilization of tumor blood vessels and promotion of tumor growth [71]. Thus, the SVF from adipose tissue is a rich source of tumor-promoting cell types, which the cancer cells can co-opt to further their progression.
Adipocytes
Not all adipocytes are identical—while the image of “white adipose tissue” comes to mind when the word “fat” is mentioned, research has uncovered at least three different types of adipocytes, each with distinct biology and functions, namely, “white,” “brown,” and the reversible “beige.” In addition, the anatomical location of the adipose tissue also determines the proportion of various “colored” adipocytes and their overall systemic function [72].
Types and Functions of Adipocytes
Classic white adipocytes , their color being reflective of their high lipid content, represent the majority of the cells in visceral and subcutaneous adipose tissue depots, which are areas that expand with obesity [68]. They are the predominant cell type involved in energy storage and release of hormones and other factors such as cytokines (see the section “Adipose-Derived Factors and Cancer” of this chapter), and they have a unilocular lipid droplet structure [72, 73]. Brown adipocytes, on the other hand, have high mitochondria content and thus an abundance of cytochromes, which gives the tissue a “brownish” tint. They are characterized by multilocular lipid droplets and are involved in non-shivering thermogenesis, where fatty acids within the cells undergo beta-oxidation, followed by the uncoupling of electron transport from ATP production, thereby creating heat. This uncoupling is carried out by uncoupling protein 1 (UCP1) located in the mitochondrial membrane [73]. As such, in highly generalized terms, white adipocytes are characterized as being “UCP1 low” and involved in metabolic responses, whereas brown adipocytes are “UCP1 high” and involved in temperature regulation.
Until recently, brown adipocytes were thought to be limited to, at least in humans, neonates that have not acquired the ability to shiver and generate body heat. However, adipose tissue with both metabolic and thermogenic activities in human adults was recently reported [74]; subsequent research suggests that white adipocytes can undergo what is known as a “browning” process, forming “beige cells” [75]. These beige cells, also known as “brite” (brown in white) cells, are similar to brown adipocytes in the sense that they have multilocular lipid droplets and express a handful of other brown fat-specific genes [76]. However, they resemble white adipocytes as they have low basal levels of UCP1, which can be stimulated (e.g., through cold adaptation) to the levels observed in bona fide brown adipocytes [77]. In contrast, beige adipocytes can also undergo a “whitening” process , whereby warm adaptation causes them to change their morphology and upregulate white adipocyte-specific genes [78]. Recent research suggests that brown and white adipocytes arise from distinctive precursor cells—brown adipocytes are derived from Myf-5+ myogenic lineage, whereas white adipocytes are derived from adipogenic precursors [79, 80]. Beige cells, on the other hand, may arise from adipogenic precursors (as a result of transdifferentiation from white adipocytes [81, 82]). However, this may not be the primary source, as demonstrated by lineage tracing methodology [83]. Skeletal muscle-residing precursor cells [84], or bipotential precursor cells expressing platelet-derived growth factor receptor alpha (PDGFRα), can differentiate directly into white or beige adipocytes depending on the stimulus [85].
In the following section, we will focus on the role of white adipocytes in cancer, as these are the major cell types in the usual adipose depot surrounding an expanding tumor. Additionally, white adipocytes are prominent secretory cells, secreting a diverse range of molecules including, but not limited to, adipokines, growth factors, hormones, ECM proteins, signaling molecules, and free fatty acids (Table 6.1, adapted from [73] and Table 5.3 of [86
]); this highlights the potential for white adipocytes as an important and pervasive cell type within the TME.
Table 6.1
List of adipose tissue-derived factors
Metabolism Apolipoprotein E (ApoE) Autotaxin Desnutrin Free fatty acids Glycerol Lipoprotein lipase Lysophosphatidic acid Zinc-a2-glycoprotein (ZAG) Steroid/hormones Estradiol Estrone Resistin Testosterone Growth factors/cytokines Fibroblast growth factors (FGF) IL-1 IL-6 IL-8 IL-10 IL-17 IL-18 Insulin-like growth factor I (IGF1) Lipocalin 2 Macrophage migration inhibitory factor (MIF) Nerve growth factor (NGF) Tumor necrosis factor (TNFα) Vascular endothelial growth factor (VEGF) Tissue factor (TF) Transforming growth factor beta (TGFβ) Chemokines CC-chemokine ligand 2 (CCL2/monocyte chemotactic protein 1, MCP1) CC-chemokine ligand 7 (CCL7) C-reactive protein (CRP) CXC-chemokine ligand 5 (CXCL5/RANTES) Vasoactive factors Adipocyte-derived relaxing factor Angiotensinogen Angiopoietin-1 Angiopoietin-2 | Angiopoietin-related protein 4 (Angiopoietin-like 4/Fasting-induced adipose factor) Angiotensin II Atrial natriuretic factor Monobutyrin Eicosanoids Prostacyclin (Prostaglandin I2) Prostaglandin E2 Prostaglandin F2α Complement system Complement factor B Complement factor C Complement factor C1q Complement factor C3 Acylation-stimulating protein (ASP/C3adesArg) Complement factor D (Adipsin) Binding proteins A1-acid glycoprotein Apelin Ceruloplasmin Cholesterol ester transfer protein (CETP) Haptoglobin Insulin-like growth factor mRNA-binding proteins Intercellular adhesion molecule 1 (ICAM1) Metallothionein Osteonectin Pentraxin family member 3 (PTX3) Retinol-binding protein Serum amyloid A (SAA) Tumor necrosis factor receptors Vascular cell adhesion molecule 1 (VCAM1) Extracellular matrix Collagen IV Fibronectin Matrix metalloproteinases (MMP) MMP1 MMP3 MMP7 | MMP9 MMP10 MMP11 MMP14 MMP15 Adipokines Adiponectin Leptin Other Adipose-tissue derived factors Adipose-specific fatty acid-binding protein (a-FABP) Adipophilin (perilipin 2) Agouti protein Chemerin High mobility group box 1 (HMGB1) Intelectin-1 (omentin) Irisin Plaminogen activator inhibitor 1 (PAI1) Resistin-like molecules (RELM) Secreted frizzeled-related protein 5 (SFRP5) Visfatin (PBEF/NAMPT) Vaspin |
Adipocytes and Cancer
As adipocytes mature from precursor cells, there is an increase in the secretion of basement membrane ECM proteins such as laminins and type IV, V, and VI collagens [87–89]; as such, normal mature adipocytes are generally separated from epithelial cells by basement membranes. During normal body homeostasis, such as mammary gland involution, the separation between cells is disrupted for the extensive restructuring of the gland. This breaking down of basement membranes and co-mingling of different cell type mimics the process of invasive breast tumors [90]. It is likely that during tumor expansion and invasion, cancer cells will first come into contact with adipocytes. As adipocytes are fragile cells with thin membranes, these physical interactions may induce injury to the adipocytes (i.e., “squeezed” by the expanding tumor cells), causing rupture or damage to the adipocyte membranes, thus releasing their cellular contents, which in turn induces inflammatory responses in the tumor site [91, 92]. Indeed, recent research demonstrated that tumor cells actively alter the adjacent adipose tissue, whereby the size of adipocytes is reduced, indicating lipolysis and modification of both intracellular lipid droplets and basement membrane components surrounding the adipocytes [91, 93, 94]. Extensive fibrosis in the tumor-associated adipose tissue was also evident using Picrosirius Red stain, and the release of lipids/triglycerides into the TME as a result of adipocyte injury was suggested to trigger macrophage activation and inflammation [91, 95]. This release of lipids and triglycerides may have a twofold effect—in addition to recruitment of immune cells, these molecules may be taken in by cancer cells as an energy source, thereby accelerating tumor growth [96].
Injury to the adipocytes may also in turn lead to necrotic cell death, causing release of damage-associated molecular patterns (DAMPs) , such as the nonhistone chromatin-associated protein high mobility group box 1 (HMGB1), as well as pro-inflammatory cytokines such as IL-6 into the TME [91, 92]. Peritumoral adipose tissue shows an extensive inflammatory response and an increase in macrophages, which may be derived from proliferating resident macrophages within the SVF or mobilized from other sources such as the circulation [92]. Thus, through recruitment of pro-tumor immune cells, tumor-associated adipocytes exacerbate tumor-associated inflammation in the TME and promote tumor progression. This is perhaps best demonstrated by studies showing that when tumors are implanted in sites distant from adipose tissues, they show a growth delay compared to those implanted in adipose-rich areas [92, 97].
In addition to physical injury, there may also be pro-tumor responses due to heterotypic cross talk between tumor/stromal cells and adipocytes. It is well-established that tumor cells release enzymes that modify the ECM [12, 13]; as these factors chew through the basement membrane, the once-sequestered adipocytes can now interact with other cell types (including tumor cells) within the adipose depot and the TME. While it is challenging to differentiate between the effects of adipocytes on other cells and vice versa in vivo, one can postulate that tumor-educated adipocytes elicit very different responses from naive adipocytes one example would be the increased angiogenesis in tumor-associated adipose tissues compared to control adipose tissues—when placed in ex vivo culture [92]. Thus, heterotypic cross talk between cancer cells and adipocytes appears to favor tumor progression as co-injection of murine adipocytes and human breast cancer cells demonstrated that adipocytes promote tumorigenesis [98]. Mature adipocytes , but not pre-adipocytes, also promote breast cancer proliferation in matrix [99], whereas in colon cancer both pre-adipocytes and mature adipocytes promote proliferation [100]. Adipocytes were also shown to increase prostate cancer cell proliferation [101] and migration [62], as well as promote pancreatic cancer in mouse models [102]. Taken together, these results clearly demonstrate a pro-tumorigenic role for adipocytes in many different contexts.
Using an experimental metastasis model, cancer cells previously exposed to adipocytes during culture prior to tail vein injection yielded more lung metastases than those cultured without adipocytes [103]. In the same study, the authors also demonstrated that cancer cells co-cultured with adipocytes, or in adipocyte-conditioned media (from both normal and cancer-associated adipocytes), have higher in vitro invasive capabilities [103]. Similarly, migration/invasion as well as wound-healing abilities of the cancer cells are increased after co-culture with adipocytes [89], and adipocyte-conditioned media promotes breast cancer cell proliferation [98]. As the cancer cells were not in direct contact with adipocytes in the latter experiments, it is suggested that this increase in invasion is mediated through secreted factors from adipocytes and does not require direct cell-cell contact. Co-culture experiments also demonstrated that adipocytes elicit a protective effect on breast cancer cells against irradiation, through the activation of Chk1 and prevention of cell death. The authors also described an increase in IL-6 expression in tumor cells after co-culture with adipocytes, which may be the mediator underlying this radioresistant phenotype [104]. Thus, it is evident that adipocyte-derived factors are important in mediating multiple aspects of tumor progression, including invasion and responses to therapy.
Adipocytes are among the first cell types that interact with emerging tumor cells; thus it is not surprising that adipocytes may be diminished even at an early stage of tumor development. Research has demonstrated a dynamic desmoplastic response elicited by adipocytes at the edge of the tumor, where adipocytes are shown to be high in abundance. As the focus is shifted toward the tumor center, adipocytes are no longer detected, and instead CAFs are in abundance [93, 103]. Furthermore, adipocytes co-cultured with cancer cells in vitro undergo extensive phenotypic changes, showing an elongated fibroblast-like cell morphology [89, 103], as well as a decrease in adipocyte marker expression and an increase of proteases and inflammatory cytokines [103]. Peritumoral adipose tissue also displays atrophied adipocytes with a decrease in expression of adipocyte-specific markers [91]. These results raised the possibility that tumor-educated adipocytes may dedifferentiate to form other pro-cancer stromal cell types that promote cancer progression.
Adipocytes are derived from mesenchymal precursors and are believed to be postmitotic. Though it was suggested that adipocytes juxtaposed to tumors could dedifferentiate and reenter the cell cycle, it has been challenging to rule out interference from other stromal cell types such as pre-adipocytes or fibroblasts [70, 71, 103]. Previous research has suggested “transdifferentiation” between white and beige adipocytes, so it is plausible that mature adipocytes, when under the right stimulus, could dedifferentiate into other cell types. This is supported by studies that showed adipocytes co-cultured with cancer cells acquire fibroblast-like features [103]. Notably, Bochet et al. demonstrated that in athymic nude mice with engrafted GFP-expressing adipose tissues containing breast cancer cells, there is an emergence of GFP-expressing stromal cells expressing FSP-1 (a marker for fibroblasts) within the tumors. Interestingly, these green stromal cells do not express the classical CAF marker α-SMA [105]. They also showed that in human breast cancer specimens, there is an increase of FSP-1 expressing cells as the focus shifts from the adipose tissue surrounding the tumor toward the tumor core [105]. With an in vivo lineage tracing model whereby all mature adipocytes are indelibly marked in an immunocompetent mouse (the adipoq-Cre:ZSgreen mice, where the ZSgreen fluorescent protein is produced in adiponectin-driven Cre-expressing cells, i.e., adipocytes [106]), and implanting syngeneic cancer cells, one could precisely follow the progression of adipocytes as tumors develop and explore how cancer cells impact the differentiation of adipocytes in vivo. Recently, we have injected syngeneic murine mammary carcinoma cells into the mammary fat pad of immunocompetent adipoq-Cre:ZSgreen mice and showed the presence of green fluorescent cells with a spindle-shaped morphology within the tumor core (Fig. 6.2). These preliminary findings indicate that as tumors progress, the adipocytes (the honeycomb-like structures surrounding the tumor) incorporated into tumors change their morphology. Future studies will be needed to elaborate how the function of these spindle-shaped, adipocyte-derived cells might differ from their mature adipocyte counterparts in tumors and what role they play during tumor growth.


Fig. 6.2
Co-option and de-differentiation of adipocytes in mammary tumors. E0771 murine mammary carcinoma cells (1 × 106) were injected orthotopically into the 4th mammary fat pad of 8-week old adipoq-Cre:ZSgreen female mice. Tumors grew for a week before mice were sacrificed and tumors (together with the surrounding mammary fat pad) were collected, fixed, frozen, and sectioned. 15 μm sections were cut and nuclei were counterstained with DAPI. Tile-scans of the whole section were carried out using confocal imaging at 10× magnification. Left: tile-scan of DAPI staining of a tumor with surrounding connective tissues. The tumor is outlined with dotted white lines. Right: the same section with green fluorescence. Green cells are adiponectin-expressing adipocytes. As seen from the image, peritumoral adipocytes (green cells) have a typical honeycombed appearance. Within the tumor, individual green cells are observed that were likely co-opted as the tumor expanded (marked with asterisks). Towards the center, green cells with a spindle morphology can be clearly observed. Inset: higher magnification of the tumor core (40×) showing the adipocyte-derived spindle cells
In addition to the role of adipocytes in promoting tumor growth and invasion/metastasis at the primary tumor site, adipocytes residing in secondary organ sites may also promote cancer progression by providing a favorable environment for cancer cells that have disseminated from the primary site. One good example would be the bone marrow (BM), which is a prime metastatic target site for many cancers such as multiple myeloma, breast, and prostate cancers. Brown et al. first showed that prostate cancer cells are attracted to adipocytes residing within the metabolically active red bone marrow [107, 108]. More recently BM adipocyte-derived factors CXCL1 and CXCL2 were shown to promote osteolysis in prostate cancer, in turn facilitating metastatic colonization of the cancer cells [109]. BM adipocytes also promote metastatic tumor growth in breast and prostate cancer through upregulation of FABP4, IL-1β, and HMOX1, which in addition to their effects on proliferation also promote the invasiveness of the disseminated cancer cells [110].
Adipose-Derived Factors and Cancer
Adipokines and Cancer
As summarized in Table 6.1, adipose tissue secretes a wide range of molecules; for some of the mentioned molecules—collectively known as “adipokines”—the adipocytes are the predominant source. Here, we will focus on the two major adipokines and discuss their role in cancer progression.
Leptin
Leptin is a 16-kDa protein encoded by the Ob gene and is predominantly produced by white adipose tissue. There are also other sites of production, albeit at vastly lower quantities, including the placenta, ovaries, bone marrow, intestine, stomach, pituitary gland, liver, brain, mammary epithelial cells, and skeletal muscle [111]. As leptin is produced by white adipocytes, its expression is positively correlated with body mass. However, leptin production is also controlled by levels of various factors, such as insulin, tumor necrosis factor alpha (TNFα), glucocorticoids, sex hormones, prostaglandins, as well as hypoxia (low oxygen levels, commonly observed in solid tumors) through HIF-1 regulation [112, 113]. Leptin controls satiety and regulates energy homeostasis by acting on the arcuate nuclease of the hypothalamus [114]. The leptin receptor has six isoforms (Ob-Ra, Ob-Rb, Ob-Rc, Ob-Rd, Ob-Re, Ob-Rf), which are from the extended class I cytokine receptor family and dimerize with one another without intrinsic kinase activity [115]. The receptor isoform expression varies according to tissue/cell type and is autoregulated by ligand stimulation [116, 117]. After binding to the receptors, leptin activates various signaling pathways such as the JAK/STAT, MAPK, PI3K (also known as AKT), AMPK, and IRS pathways [118–120].
Previous research has shown that leptin promotes proliferation, migration, and invasion in tumor cells, identifying it as a cancer-promoting factor [112, 121]. Leptin promotes proliferation of prostate cancer through the activation of MAPK, PI3K, and JNK/MAP kinase pathways [122]. It also promotes proliferation of breast cancer cells and colorectal cancer cells through the activation of JAK/STAT3, MAPK-ERK1/2, and PI3K pathways [123–125]. Leptin trans-activates ErbB2 and EGFR and interacts with IGF-1, thereby promoting invasion and migration. It also increases estrogen levels by stimulating aromatase expression and can directly activate the estrogen receptor (ER), thus further promoting the growth of ER-positive breast cancer cells [126–128]. Apoptosis is also inhibited by leptin in colorectal cancer [123–125], and invasion is increased by leptin-promoted proteolytic cleavage of ECM [129]. In addition to its effects on cancer cells directly, leptin stimulates inflammatory cytokine (e.g., IL-6, TNFα) production in macrophages, i.e., favoring the tumor-promoting M2 subtype [130]. Additionally, leptin has been shown to regulate endothelial cell proliferation and increase endothelial COX-2 expression [131, 132]; it also promotes angiogenesis in prostate and colorectal cancers by inducing the expression of VEGF, FGF2, MMP2, and MMP9 [133, 134] and increases the expression of VEGF-receptor 2 (VEGF-R2), further eliciting an angiogenesis-promoting effect in breast cancer [135].
Adiponectin
Adiponectin is a 30-kDa protein that belongs to the complement-1q family [136]. It is primarily secreted in the monomeric form but can further oligomerize to form trimeric, hexameric, or multimeric forms [111]. It can also be cleaved by leukocyte elastase, generating a globular oligomeric complex [137]. Adiponectin is expressed by adipocytes in high concentrations and is generally thought of as adipocyte specific, although studies have reported expression in the skeletal muscle, liver, colon, salivary glands, bone marrow, fetal tissue, placenta, and cerebrospinal fluid [138], albeit at much lower levels. Paradoxically, even though adiponectin is predominantly expressed by adipocytes, the circulation levels are negatively correlated with BMI and body fat percentage, which is likely due to negative regulation by various other adipose-secreted proteins, such as TNFα, IL-6, and IL18 [139, 140]. There are two main receptors for adiponectin: adiponectin receptor 1 (adipoR1) and adiponectin receptor 2 (adipoR2). AdipoR1 preferentially binds to globular adiponectin, whereas adipoR2 binds to other higher-molecular weight forms more readily [141]. These receptors then either homo- or heterodimerize and activate downstream signaling events through phosphorylation, such as AMPK [142, 143], acetyl-CoA carboxylase [144], and MAPK [141]. Adiponectin is an insulin sensitizer [145] and has anti-atherogenic [146] and anti-inflammatory [147] effects. It also maintains the vasculature within adult adipose tissue and is likely an angiogenesis inhibitor [148].
In general, adiponectin and leptin have opposing effects on cancer cells and can antagonize one another, e.g., adiponectin suppresses leptin-induced IL-6 signaling, by inhibiting autocrine IL-6 production, decreasing soluble IL-6 receptors, and increasing sgp130 (inhibitor of the IL-6/IL-6receptor complex) [149, 150]. Where leptin administration increases proliferation, migration, and invasion of cancer cells, adiponectin treatment in mouse models was shown to inhibit tumor growth by decreasing proliferation and increasing apoptosis of cancer cells; it also inhibits invasion of cancer cells and leads to decreased vessel density in tumors [151–155]. As such, adiponectin is widely viewed as an antitumor factor. Adiponectin was shown to inhibit proliferation of prostate cancer cells through androgen receptor-dependent (inhibition of STAT3 signaling [156]) or androgen receptor-independent mechanisms (activation of AMPK and thus inhibition of mTOR/PI3K/AKT pathways [157]). It also inhibits TNFα in breast cancer cells, thereby decreasing estrogen synthesis and inhibiting NFκB signaling, leading to a decrease in proliferation [158]. This inhibition of TNFα also suppresses VEGF production, in turn causing a decrease in angiogenesis and subsequent increase in apoptosis/necrosis within the tumor mass [148, 157]. The effects of adiponectin in endometrial cancer is, unsurprisingly, similar to that observed in breast cancer, as both tissues respond to estrogens and express high concentrations of VEGF [111, 159]. In addition, adiponectin can induce cell cycle arrest and apoptosis through PTEN-dependent (PI3K/Akt) or PTEN-independent (Erk1/2 and cyclin E2) mechanisms in endometrial cancer [160]. In terms of colorectal cancer, adiponectin also has antiproliferative and pro-apoptotic effects through the activation of AMPK and inhibition of the mTOR pathway [161].
Other Adipose Tissue-Derived Factors and Cancer
In this section, we will discuss a few other adipose tissue-derived factors and their roles in cancer. These molecules are frequently grouped together with adiponectin and leptin as “adipokines”; however, as these molecules may not be predominantly secreted by adipocytes, but rather derived from the adipose tissue (which includes the SVF), we refer to them here as “adipose tissue-derived factors” instead. Many of these factors have intricate relationships with the aforementioned adipokines. Additionally, as briefly discussed in the previous section, injured adipocytes likely passively release factors (as opposed to “secrete,” which suggests an active and controlled process) that fuel tumor progression.
Visfatin/PBEF/NAMPT
Visfatin , a 52-kDa protein, is also known as pre-B-cell colony-enhancing factor (PBEF) or nicotinamide mononucleotide denylytransferase (NAMPT) . It was first identified as PBEF due to its effects on the development and maturation of B-lymphocytes [162]. It was then identified as an adipokine that is highly expressed in visceral fat, with circulating levels correlating with the size of the visceral fat depot (but not subcutaneous fat) and thus was termed “vis(ceral)fat” in [33, 163]. Fukuhara et al. also described it as a hormone that binds and activates the insulin receptor, thereby mimicking insulin signaling. However, this study was retracted in 2007, throwing the physiological relevance of visfatin into question. Nonetheless, in a later study, it was described as NAMPT, a key enzyme involved in NAD biosynthesis from nicotinamide [164], thus considerably expanding its biological implications beyond adipose tissue. Additionally, visfatin was shown to be released predominantly from macrophages rather than adipocytes residing in visceral adipose tissues [165] (although visfatin circulation levels are usually increased in obesity), as such visfatin is involved in inflammation. The pro-angiogenic effects of visfatin are also well documented [166], which occurs through Erk1/2 activation [167], VEGF/MMP2/MMP9 production [168], FGF-2 upregulation [169], and MCP1/CCR2 induction [170].
In terms of visfatin and cancer, it was shown that visfatin inhibition confers greater sensitivity toward chemically-induced apoptosis in fibrosarcoma cells [171], and exogenous expression of visfatin in prostate cancer increases proliferation through Erk1/2 and p38, as well as the expression of MMP2/9, suggesting another link with increased invasive capabilities [172]. Similarly, the inhibition of visfatin suppresses in vitro proliferation and in vivo tumor growth of prostate cancer cells, with a further sensitization of the cancer cells toward chemotherapeutic treatment [173]. Visfatin was also demonstrated to promote proliferation through Notch1 signaling in breast cancer cells [174]. Of note, one known small molecule inhibitor of visfatin, FK866/APO866, is being evaluated as a cancer therapeutic [166] and has completed Phase II clinical trials. Another, CHS828/GMX1777, despite promising preclinical results [166], could not complete clinical trials due to financial constraints.
PAI-1
Plasminogen activator inhibitor-1 (PAI-1) is a serine protease inhibitor that is secreted by endothelial cells, stromal cells, and visceral WAT [175]. It affects adipocyte differentiation and insulin signaling, and circulating levels are positively associated with obesity as a result of increased PAI-1 production in obese adipocytes [176]. PAI-1 inhibits urokinase-type and tissue-type plasminogen activators (uPA and tPA), thereby blocking fibrinolysis and remodeling of the ECM. PAI-1 both promotes and inhibits angiogenesis depending on the concentration [177]; therefore, its expression plays a critical role in tumor growth, invasion, and metastasis [178]. uPA expression is positively associated with tumor growth, invasion, and metastasis [179], whereas PAI-1, an inhibitor of uPA, is associated with both anti- and pro-cancer activity.
PAI-1 was reported to inhibit prostate tumor growth, angiogenesis, invasion, and metastasis [180] and appeared to negatively impact mammary adenocarcinoma progression [181]. However, PAI-1 was also shown to promote tumor invasion and angiogenesis, where host-derived PAI-1 is more important than tumor-secreted PAI-1 [182]. Additionally, PAI-1 treatment conferred protection against chemotherapeutics in prostate cancer and leukemia cell lines, through inhibition of apoptosis [183]. Loss of PAI-1 also reduces tumor growth, invasion, and metastasis in fibrosarcoma and non-melanoma skin cancer [184, 185]. The contrasting results suggest that the effects are contextual based on the levels of PAI-1—where low levels (nearer physiological levels) increase angiogenesis and elevated levels does the opposite [179]. However, the effects of PAI-1 on cancer progression are not restricted to angiogenesis—PAI-1 interacts with vitronectin [186] and integrins, thus regulating adhesion [187] and migration properties of cancer cells [188]. It is also implemented in neutrophil recruitment and the inflammation response [189]. Thus, PAI-1 both positively and negatively mediates tumor progression depending on the context and tumor type.
TNFα
Tumor necrosis factor alpha (TNFα) is a 25-kDa cytokine that plays an important role in the adaptive immune system. It is a transmembrane protein with signaling potential both as a membrane-integrated protein and as a soluble cytokine released after proteolytic cleavage (see [190] for a detailed review). TNFα is a key growth factor that is secreted by macrophages, although adipocytes are also known to secrete it [191], and TNFα levels are elevated in obese individuals, again likely reflecting a chronic inflammatory state [59, 192]. It has two receptors: TNFR1, which is found in most cell types and activated by soluble TNFα, and TNFR2, predominantly found on hematopoietic cells that preferentially bind the transmembrane TNFα. Antitumor effects of TNFα were reported in gastric cancer, where it activates caspase-3 and thus apoptosis [193], and TNFα was thought to be a strong candidate as a cancer therapeutic as its name suggested [190]. However, as research demonstrated that TNFα is not only produced by cancer cells but also present in high amounts in the TME, it is increasingly clear that TNFα can promote carcinogenesis, likely through the activation of NFκB, which in turn promotes the expression of factors that stimulate inflammation, proliferation, survival, invasion, and metastasis [194, 195]. TNFα further fuels cancer progression by inducing the production of other pro-cancer cytokines, angiogenic factors, and MMPs, thereby promoting growth and invasion of tumor cells [196]. Furthermore, using dietary/genetically induced obese mouse models, it was shown that liver inflammation and tumorigenesis are enhanced through increased TNFα and IL-6 expression [59], further highlighting the link between “disease state adipocytes,” adipose-derived factors, and tumor-promoting activities. As such, TNFα is an attractive therapeutic target as it promotes tumor progression on several levels. TNFα has tumor-cytotoxic properties in certain contexts; however, it requires careful administration to ensure direct contact with the cancer cells, in order to obtain maximal benefit [190]. On the contrary, TNFα inhibitors showed some success in clinical trials for various cancers and may be considered good candidates as combination therapies either by increasing sensitivity to chemotherapy [197, 198] or by re-education of the TME [199].
IL-6
Similar to TNFα, interleukin-6 (IL-6) is a well-known inflammatory cytokine that is secreted by macrophages as well as adipocytes (albeit in lower amounts) and is elevated in obese patients [59, 192]. Additionally, IL-6 expression levels are elevated in cancer-associated adipocytes [91, 103]. The role of IL-6 in cancer has been debated, as IL-6 was shown to be both pro- and anti-apoptotic in breast cancer cells [200–203]; nonetheless, IL-6 is generally thought of as pro-cancer, where IL-6 treatment induces malignant features in mammospheres from ductal breast carcinoma as well as normal mammary gland [204]. IL-6 induces the production of many proangiogenic molecules including VEGF, which contributes to tumor growth and metastasis through the angiogenic switch [205]. It also activates/increases aromatase expression involved in estrogen synthesis and therefore is likely to have a major role in cancer progression in postmenopausal female patients [202]. IL-6 knockout mice demonstrated a resistance toward carcinogen-induced tumorigenesis in liver cancer [204], and IL-6 has been shown to increase the transcription factor Jagged-1, in turn enhancing self-renewal capabilities of breast cancer stem-like cells [111]. As mentioned above, IL-6 is upregulated in obese mice with liver cancer, again potentially linking adipocytes with tumor-promoting functions [59].
Diagnostic Value of Adipocytes in Cancer
Based on in vitro and in vivo evidence, adipocytes appear to be important in mediating cancer progression in the TME. But how do adipocytes in the TME impact clinical outcomes? Studies have been conducted to evaluate the prognostic values of local adipose tissue invasion by cancer cells at the tumor edge and reported a correlation between high adipose tissue invasion with poor patient outcomes for breast, prostate, pancreas, kidney, and colon cancers [206–208]. Histological studies on steatosis (adipocyte infiltration of the liver) and adipocyte infiltration of the pancreas have both been linked to an increased risk of hepatocellular and pancreatic cancers, and in terms of established cancers, adipocyte infiltration in pancreatic cancer and breast cancers are both associated with increased aggressiveness and faster disease progression [208, 209].
Interestingly, IL-6 expression in tumor-surrounding adipocytes had been shown to be elevated in human breast cancer patients with higher tumor grade and/or lymph nodes involvement; similarly, this increase of IL-6 in tumor-surrounding adipocytes was also observed in prostate cancer [210]. These results suggest that in addition to detection of adipocyte infiltration into tumor tissues, assessing the levels of adipose-derived factors in tumor-associated adipose tissue may also be prognostic. All of these studies using human patient samples highlight the feasibility of using adipocytes to assess cancer incidence, as well as predict disease outcome and progression.
Diagnostic Value of Adipose-Derived Factors in Cancer
Circulating Adipokines/Adipose Tissue-Derived Factors
Many of the adipocyte-derived factors are influenced by a multitude of other soluble factors, which may be highly variable between patients (e.g., estrogen levels); in addition, these factors also influence the activity and/or expression of one another (e.g., leptin, adiponectin, TNFa, IL-6). Thus, the effectiveness of studying the serum levels of adipokines in cancer patients is often complicated, and contradictory reports in correlating serum levels of adipokines with cancer progression is not unusual.
High serum leptin levels have been associated with increased risk for colorectal cancer [211], but mixed reports exist [212–217], where higher leptin levels may be indicative of better prognosis [218]. In terms of breast cancer, after unifying contradictory results in case-control studies, three out of ten studies showed positive correlation between leptin levels and breast cancer, while the others showed no association [219]. In this instance, it is challenging to draw a conclusion on the relationship between leptin and breast cancer stage, as the various studies did not have a unified study design (e.g., sample collection from fasting/non-fasting patients, stratification of women into premenopausal or postmenopausal statuses, history of leptin-related diseases such as diabetes, etc.). Interestingly, where the three studies showing positive correlation between serum leptin and breast cancer regardless of menopause stage, one study showed that in premenopausal patients, an inverse association of leptin and breast cancer was reported [220]. This result was also found in a later study where postmenopausal breast cancer patients showed a correlation between high leptin levels and tumor grade/stage [221], thus indicating that postmenopausal women may be particularly affected by leptin, likely due to the increased importance of the adipose tissue as a source of estrogens [221, 222]. A small study in Turkish patients indicated that higher circulating leptin levels are observed in patients with papillary thyroid carcinoma [223], but more studies need to be conducted to confirm this finding. Surprisingly, in a small case-control study on patients with renal cell carcinoma, leptin was inversely associated with cancer risk [224]; however, there was no adjustment for sex or tumor stage. In a later study with a much larger patient cohort, a significant association between high leptin concentration and increased renal cell carcinoma was observed, although the authors also conceded this correlation may be dependent on the racial background [225]. Furthermore, higher leptin serum levels were also shown to be a predictor for shorter progression-free survival [226].
Adiponectin levels are inversely related with risk, incidence, and tumor grades of prostate cancer [227], but its relationship with female breast cancer is dependent on the menopausal state, where a correlation between low adiponectin levels and breast cancer is only observed in postmenopausal women [228]. In contrast, in endometrial cancer, a link between low circulating adiponectin levels and cancer risk is strongly represented in premenopausal women, independent of BMI status [159, 229]. Contradicting results have been reported between adiponectin and colorectal cancer [214, 230], although low circulating adiponectin is mostly associated with an increase in colorectal cancer incidence [231–233]. Conversely, in pancreatic cancer, case-controlled studies suggest that increased circulating adiponectin levels are associated with cancer incidence [234, 235], although in male smokers, an opposite trend was reported [236]. Low circulating adiponectin is also strongly associated with renal cancer incidence, with a further inverse correlation between adiponectin levels and occurrence of metastases; this is despite a lack of consistency between adiponectin levels and tumor grade [237–239]. In addition, in patients with end-stage renal disease, who have a higher risk of cancer incidence, low circulating adiponectin levels are an independent predictor of malignancy [240]. This suggests that in other chronic diseases that have a link with cancer incidence (e.g., Crohn’s disease), circulating adiponectin levels may also be useful as a malignancy predictor.
Serum levels of visfatin were shown to be significantly higher in endometrial cancer patients and are correlated with visfatin expression within the tumor [241]. Additionally, in colorectal cancer patients, high circulating visfatin levels were identified as a significant risk factor for both early and advanced diseases, and levels were positively correlated with tumor stage progression [214, 242]; a similar trend was reported in gastric cancer patients [243], further confirming a strong clinical relevance for visfatin as a biomarker of cancer incidence and progression.
For the diagnostic value of TNFα, high circulating levels of TNFα and its soluble receptor (in particular sTNFR2) were shown to be correlated to a higher risk of endometrial cancer in a case-control study [244], and TNFα levels in blood are also positively correlated with disease stage and severity in prostate cancer [245, 246].
Increased serum IL-6 levels are linked to a significant increase in endometrial cancer risk [247], and interestingly, one potential reason why IL-6 may contribute to endometrial carcinogenesis in postmenopausal women is through adipocytes, where an increase in adipocyte-derived estrogen as a result of IL-6 stimulation drives hyperplasia and ER-positive tumor growth [248]. Circulating IL-6 levels are also positively correlated with tumor grade and worse overall outcome in prostate cancer patients [245, 249], multiple myeloma [250], metastatic renal cell carcinoma [251] and breast cancer [205, 252, 253] and are identified as a risk factor for colorectal cancer in postmenopausal women [211]. Interestingly, it was reported that reduced IL-6 or sIL-6R (soluble IL-6 receptor) levels in the serum of patients indicate a response to therapy [254], suggesting the potential of using adipose-derived factor levels as an indicator for treatment response.
Studies in breast cancer demonstrated that a combination of both leptin and adiponectin circulating levels (i.e., ratio between the two adipokines) may be a better prognostic predictor for patient outcome [255–258]. This highlights the complex relationships between various adipokines and adipose tissue-derived factors and suggests a multifactorial analysis where all of these factors must be taken into account in order to utilize them as prognostic indicators.
Expression of Adipokines/Adipose Tissue-Derived Factors in Tumor Tissues
Despite the s hortcomings of using circulating levels as a biomarker for cancer progression, adipokines/adipose tissue-derived factors may still be used as prognostic markers through detection of their expression(s) in biopsy samples. For example, leptin and its receptor are overexpressed in human primary and metastatic breast cancer, with the highest levels detected in poorly differentiated tumors, which are usually associated with worse patient outcome [259]. Additionally, differentiating between the subtypes of leptin receptor in these breast cancer patients may also be a prognostic factor, where patients with high Ob-Ra-only have longer disease-free survival compared to patients with a high Ob-Rb/Ob-Ra expression ratio [260]. Similarly, leptin is overexpressed in colorectal cancers and is significantly correlated with tumor grade [261]. Leptin and leptin receptor expression levels in papillary thyroid cancer are positively associated with aggressiveness of the disease [262, 263], and high leptin receptor levels in renal cell carcinoma were associated with venous invasion/tumor grade/presence of lymph node metastasis [226].
In terms of adiponectin, the expression of AdipoR1 and AdipoR2 are increased in colorectal cancer cells [264]. However, in renal cancers, a decrease of receptor expression was reported suggesting that the use of AdipoR1/R2 as a diagnostic marker may be dependent on the cancer type. Visfatin expression, on the other hand, is positively correlated with stage and myometrial invasion in patients with endometrial cancer [241]. High expression of visfatin in breast cancer tissues is associated with ER and PR status, as well as poor disease-free and overall survival in patients [265]. Interestingly, visfatin expression could predict poor response toward doxorubicin chemotherapy [266], and patients with high visfatin expression have decreased recurrence rates after hormone therapy (but not radio- or chemotherapy [265]), suggesting the potential of visfatin in stratifying treatment options for breast cancer patients. Similarly, PAI-1 expression can also predict treatment response to tamoxifen in recurrent breast cancer patients [267] and act as a predictor for shorter overall as well as disease-free survival in women with breast cancer [268].
Polymorphisms of Adipokines/Adipose Tissue-Derived Factors
In addition to using expression levels in tumors or surrounding tissues as an indicator for disease progression, mutations in the genes encoding adipocyte-derived factors may also be used for cancer risk assessment, similar to the BRCA1/BRCA2 mutations in breast cancer patients. Polymorphisms of leptin (LEP) and leptin receptor (Ob-R) were associated with higher risk of breast cancer in women, and the association was strongest in obese postmenopausal women [269–272]. PAI-1 promoter polymorphism (known as 4G/5G polymorphism, where the 4G allele translates into higher PAI-1 expression) has also been linked to cancer incidence/prognosis. In breast cancer patients, PAI-1 4G is increased in patients with aggressive tumor characteristics [273], and another reported that 5G/5G homozygosity is a positive prognostic marker for aggressive diseases [274], although a third study found no link between PAI-1 polymorphism and breast cancer incidence/outcome. PAI-1 4G was found to be a major contributor to early stages of oral cancer [275], and PAI-1 polymorphisms may also be good prognostic markers in high-grade gliomas [276]. TNFα-308, a polymorphic form of TNFα, is a risk factor for breast [277], gastric [278], and liver cancer [279], and a single-nucleotide polymorphism in the IL-6 promoter (in particular the 174 G/C polymorphism) has been associated with more aggressive breast cancer types [280, 281].
Conclusion
As discussed in this chapter, there is great potential for adipocytes as a histological prognostic tool in cancer patients; however, the use of circulating adipose-derived factors for prognosis requires further investigation, which may involve improved patient stratification and multifactorial analyses. Nonetheless, apart from being prognostic markers, adipokines/adipose tissue-derived factors may also be useful in measuring treatment response. It should be noted that some of the molecules discussed here are considered good therapeutic targets and some small molecule inhibitors are already in clinical trials for cancer. This highlights the importance of further exploring the role of the adipose tissue and adipocytes in mediating cancer progression and metastasis.
References
1.
Siegel RL, Miller KD, Jemal A. Cancer statistics, 2015. CA Cancer J Clin. 2015;65(1):5–29. doi:10.3322/caac.21254.PubMed
2.
Torre LA, Bray F, Siegel RL, Ferlay J, Lortet-Tieulent J, Jemal A. Global cancer statistics, 2012. CA Cancer J Clin. 2015;65(2):87–108. doi:10.3322/caac.21262.PubMed
3.
Hanahan D, Weinberg RA. Hallmarks of cancer: the next generation. Cell. 2011;144(5):646–74. doi:10.1016/j.cell.2011.02.013.PubMed
4.
Egeblad M, Nakasone ES, Werb Z. Tumors as organs: complex tissues that interface with the entire organism. Dev Cell. 2010;18(6):884–901. doi:10.1016/j.devcel.2010.05.012.PubMedPubMedCentral
5.
Kalluri R, Zeisberg M. Fibroblasts in cancer. Nat Rev Cancer. 2006;6(5):392–401. doi:10.1038/nrc1877.PubMed
6.
Xing F, Saidou J, Watabe K. Cancer associated fibroblasts (CAFs) in tumor microenvironment. Front Biosci. 2010;15:166–79.
7.
Disis ML. Immune regulation of cancer. J Clin Oncol. 2010;28(29):4531–8. doi:10.1200/JCO.2009.27.2146.PubMedPubMedCentral
8.
Roorda BD, ter Elst A, Kamps WA, de Bont ESJM. Bone marrow-derived cells and tumor growth: contribution of bone marrow-derived cells to tumor micro-environments with special focus on mesenchymal stem cells. Crit Rev Oncol/Hematol. 2009;69(3):187–98. doi:10.1016/j.critrevonc.2008.06.004.
9.
Dudley AC. Tumor endothelial cells. Cold Spring Harb Perspect Med. 2012;2(3):a006536. doi:10.1101/cshperspect.a006536.PubMedPubMedCentral
10.
Hanahan D, Coussens LM. Accessories to the crime: functions of cells recruited to the tumor microenvironment. Cancer Cell. 2012;21(3):309–22. doi:10.1016/j.ccr.2012.02.022.PubMed
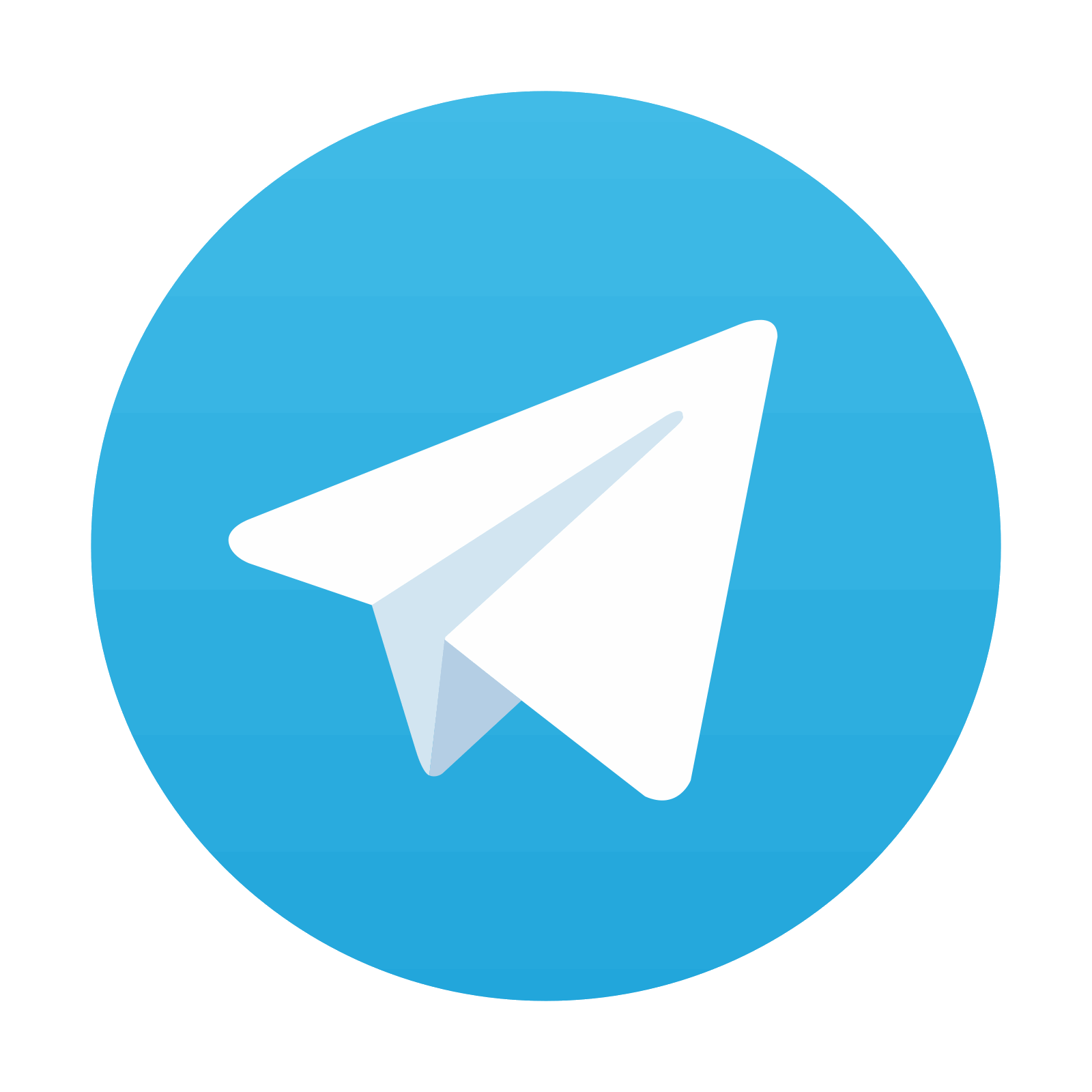
Stay updated, free articles. Join our Telegram channel
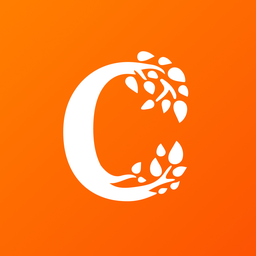
Full access? Get Clinical Tree
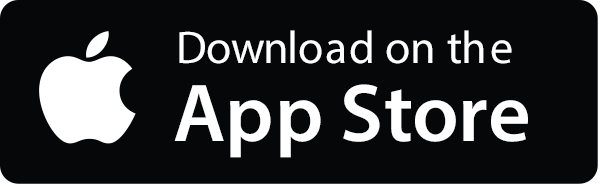
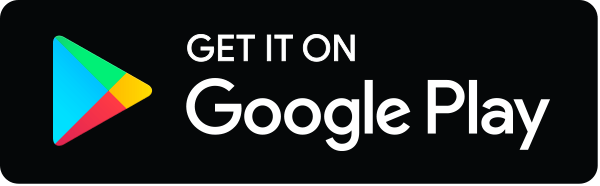