The Cardiovascular System
The final death reports for 2006 (National Vital Statistics Reports, 2009) indicate that the leading cause of death in the United States continues to be related to cardiovascular disease. According to the American Heart Association (2009), cardiovascular disease includes hypertension, coronary heart disease (myocardial infarction and angina), heart failure, stroke, and congenital heart disease. It is critical that health care providers and lay persons learn the concepts of cardiovascular disease, prevention, and health maintenance.
The cardiovascular system begins with the heart, a muscular pump that beats rhythmically and repeatedly 60 to 100 times a minute. Each beat causes blood to surge from the heart and travel throughout the body in a closed network of arteries, arterioles, and capillaries and return to the heart through venules and veins. The purpose of the cardiovascular system is to pick up oxygen in the lungs and nutrients absorbed across the gut and deliver them to all cells of the body. At the same time, the cardiovascular system removes the metabolic waste products produced by each cell and delivers them to the lungs or the kidneys to be excreted.
● Physiologic Concepts
ANATOMY OF THE HEART
The heart is a four-chambered, muscular organ that lies in the chest cavity, under the protection of the ribs, slightly to the left of the sternum. The heart sits within a loose, fluid-filled sac, called the pericardium. The four chambers of the heart include the left and right atria and the left and right ventricles.
The atria sit next to each other above the ventricles. The atria and ventricles are separated from each other by one-way valves. The right and left sides of the heart are separated by a wall of tissue called the septum. There is normally no mixing of blood between the two atria, except during fetal life, and there is never mixing of blood between the two ventricles in a healthy heart. Connective tissue surrounds all chambers. The heart is extensively innervated.
The atria sit next to each other above the ventricles. The atria and ventricles are separated from each other by one-way valves. The right and left sides of the heart are separated by a wall of tissue called the septum. There is normally no mixing of blood between the two atria, except during fetal life, and there is never mixing of blood between the two ventricles in a healthy heart. Connective tissue surrounds all chambers. The heart is extensively innervated.
TWO CIRCULATIONS OF THE CARDIOVASCULAR SYSTEM
The left side of the heart pumps oxygenated blood through the systemic circulation, which reaches all cells of the body except those involved with gas exchange in the lungs. Blood leaves the heart via the aorta through a one-way valve. The right side of the heart pumps deoxygenated blood through the pulmonary circulation, which delivers blood only to the lungs to be oxygenated. Blood leaves the right side of the heart through the pulmonary artery, which has a one-way valve.
Systemic Circulation
Blood enters the left atrium from the pulmonary vein. The pulmonary vein is the only vein in the body that carries oxygenated blood. Blood in the left atrium flows into the left ventricle through the atrioventricular (AV) valve located at the juncture of the left atrium and ventricle. This valve is called the mitral valve. All cardiac valves open when pressure in the chamber or vessel above them is greater than the pressure in the chamber or vessel below.
Blood from the left ventricle outflows into a large, muscular artery, called the aorta, through the aortic valve. Blood in the aorta is delivered throughout the systemic circulation, through arteries, arterioles, and capillaries, which then rejoin to form veins. The veins from the lower part of the body return the blood to the largest vein, the inferior vena cava. The veins from the upper body return blood to the superior vena cava. The venae cavae empty into the right atrium.
Pulmonary Circulation
Blood in the right atrium moves into the right ventricle through another AV valve, called the tricuspid valve. Blood leaves the right ventricle and travels through a fourth valve, the pulmonary valve, into the pulmonary artery. The pulmonary artery is the only artery in the body that carries unoxygenated blood. The pulmonary artery branches into left and right pulmonary arteries, which travel to the left and right lungs, respectively. In the lungs, the pulmonary arteries branch many times into arterioles and then capillaries. Each capillary perfuses past an alveolus, the unit of respiration. All capillaries reform to become venules, and the venules become veins. The veins join to form the pulmonary veins.
![]() FIGURE 13-1 Anatomy of the heart. (From Porth, C. & Matfin, G [2009]. Pathophysiology, concepts of altered health states [8th ed.]. Philadelphia: Lippincott Williams & Wilkins.) |
Blood flows in the pulmonary veins back to the left atrium, completing the blood flow cycle. The heart and the systemic and pulmonary circulations are shown in Figure 13-1.
Functions of the Systemic and Pulmonary Circulations
As blood passes each cell of the body in the systemic circulation, carbon dioxide and other cellular waste products are added to the blood, while oxygen and nutrients are delivered from the blood to the cells. In the pulmonary circuit, the opposite occurs: carbon dioxide is eliminated from the blood and oxygen is added. By continual cycling of the blood through the pulmonary and systemic circulations, oxygen supply and waste removal are ensured for all cells.
Coronary Artery Blood Flow
Two large arteries, called the left and right coronary arteries, branch off the aorta as soon as it leaves the left ventricle and supply blood to the heart. The left coronary artery quickly branches into the left anterior descending artery and the circumflex artery. Table 13-1 summarizes the portions of the heart supplied by the coronary arteries.
TABLE 13-1 Coronary Arteries | ||||||||||||
---|---|---|---|---|---|---|---|---|---|---|---|---|
|
CARDIAC MUSCLE
Cardiac muscle is composed of highly specialized muscle fibers. Not only do these fibers contract in response to action potentials produced from neural stimulation, but many cardiac muscle fibers are capable of spontaneously firing action potentials that can initiate their own contractions. Excitation-contraction of cardiac muscle is described in the next several sections, beginning with a description of the cardiac muscle fibers and finishing with an outline of how an action potential leads to the contraction and beating of the heart.
Cardiac Muscle Fibers
As described fully in Chapter 10, cardiac muscle fibers are made up of bands of protein filaments, called myofilaments, lying in series with each other. Each band is called a sarcomere. A cardiac sarcomere is shown in Figure 13-2. Sarcomeres in cardiac muscle are fused together at their borders to form areas called intercalated disks. These are areas of low resistance across which electrical currents can pass.
Myofilaments
Each cardiac sarcomere is made up of thick and thin filaments. Thick filaments consist of the contractile protein myosin. Thin filaments include the second contractile protein, actin, and two regulatory proteins, tropomyosin and troponin. Cross-bridges extend from the myosin filaments to the actin filaments. In the absence of calcium ion, tropomyosin is attached to each actin molecule in such a way that tropomyosin covers the binding site for myosin on actin, and thus inhibits cross-bridge connection. The troponin complex, which includes troponin-I, -T, and -C, is attached to the tropomyosin filament. When calcium is available, troponin-C binds the tropomyosin filament in such a way that the myosin cross-bridges are now exposed and able to bind actin.
At this point, energy stored from a previous myosin-based reaction, in which adenosine triphosphate (ATP) was split into adenosine diphosphate (ADP) and a phosphate molecule, is released and is used to swing the crossbridges. This causes the myosin and actin filaments to slide past each other and muscle contraction to occur. Because all cardiac muscle cells are connected to each other at the intercalated disks, depolarization of one group of cardiac muscle cells spreads to neighboring cells and all cells contract as a unit. Each contraction represents a heartbeat.
Cardiac Muscle Depolarization and Action Potential Firing
An action potential in a cardiac muscle cell is triggered as a result of a depolarizing current reaching it from a neighboring muscle cell. Although in some ways similar to a skeletal muscle action potential, the cardiac action potential is unique due to its plateau phase and long duration.
Just like a skeletal muscle cell, a cardiac muscle cell fires an action potential when the inside of the cell becomes significantly more positively charged than the outside. As in skeletal muscle, this occurs when voltage-sensitive sodium channels open in the cell membrane, resulting in an in-rush of positively charged sodium ions. This is called Phase 0 of the cardiac action potential and is characterized by a rapid change in the membrane potential, from about -80 mV intracellularly to a positive intracellular value of approximately 20 to 25 mV (Fig. 13-3). Very quickly, closure of the sodium channels occurs, marking the end of Phase 0. Next begins Phase 1, a repolarizing phase. During Phase 1, potassium channels close in the cell membrane and positively charged potassium ions accumulate in the cell, preventing the return of the membrane potential toward its resting (negative) value. Closure of the potassium channels immediately following the onset of the action potential does not happen in skeletal muscle; in cardiac muscle, it contributes to the slowing of repolarization. Prolongation continues during the next
phase, Phase 2, as calcium ions begin to enter the cell through calcium channels present in the cell membrane. This increase in intracellular calcium triggers the opening of other, slow calcium channels located in the membrane of the sarcoplasmic reticulum, a storage compartment for intracellular calcium. This process, called “calcium stimulated calcium release,” results in a further rise in intracellular calcium levels, reaching levels that effectively move the troponin off the tropomyosin molecule and thus trigger the muscle cell to contract. The slow inward movement of calcium also prolongs cellular depolarization. Phase 3 is the final repolarization phase, and it occurs when the influx of calcium decreases and the outflow of potassium ions through “delayed rectifier channels” begins, finally bringing the membrane potential back to its negative resting value. Intracellular levels of calcium decrease, by means of calcium moving out of membrane channels as well as via active transport of calcium back into the sarcoplasmic reticulum storage sites. As calcium falls, the cell relaxes and the heart enters diastole. The cell remains in Phase 4, the resting potential phase, until it is again stimulated to undergo an action potential. Prolongation of the action potential is significant because it makes it impossible to fire a second action potential before the first is completed, ensuring that cardiac cells will not undergo summation and tetany of contractions: If cardiac cells underwent tetany, the heart would stop pumping out blood because it would not be able to relax and fill with blood between beats.
phase, Phase 2, as calcium ions begin to enter the cell through calcium channels present in the cell membrane. This increase in intracellular calcium triggers the opening of other, slow calcium channels located in the membrane of the sarcoplasmic reticulum, a storage compartment for intracellular calcium. This process, called “calcium stimulated calcium release,” results in a further rise in intracellular calcium levels, reaching levels that effectively move the troponin off the tropomyosin molecule and thus trigger the muscle cell to contract. The slow inward movement of calcium also prolongs cellular depolarization. Phase 3 is the final repolarization phase, and it occurs when the influx of calcium decreases and the outflow of potassium ions through “delayed rectifier channels” begins, finally bringing the membrane potential back to its negative resting value. Intracellular levels of calcium decrease, by means of calcium moving out of membrane channels as well as via active transport of calcium back into the sarcoplasmic reticulum storage sites. As calcium falls, the cell relaxes and the heart enters diastole. The cell remains in Phase 4, the resting potential phase, until it is again stimulated to undergo an action potential. Prolongation of the action potential is significant because it makes it impossible to fire a second action potential before the first is completed, ensuring that cardiac cells will not undergo summation and tetany of contractions: If cardiac cells underwent tetany, the heart would stop pumping out blood because it would not be able to relax and fill with blood between beats.
Cardiac Muscle Relaxation
Adenosine diphosphate (ADP) releases from the cross-bridge after it swings, and another ATP molecule binds to the myosin protein, causing the myosin cross-bridges to separate from actin. As long as calcium ion is still available intracellularly to bind troponin, this new ATP will be split, and its energy will be released when a new cross-bridge connection forms, thereby repeating the cycle and ensuring that the muscle will continue to contract. Many crossbridge cycles and sliding of the filaments occur during one action potential. The contraction ultimately stops when intracellular calcium levels fall. Without calcium, tropomyosin again blocks the site on actin to which the crossbridges bind, and no further connection between myosin and actin can be made.
In skeletal muscle, there is always enough calcium released with each action potential to fully saturate all troponin sites and thus cause all cross-bridges to swing. In cardiac muscle, however, less than maximal amounts of calcium are usually released with a single action potential. This means that under certain circumstances, cardiac contractile strength may be increased if necessary.
The Pacemaker
Most cardiac muscle cells have the capacity to fire action potentials independently. However, certain cells are more permeable to sodium ions at rest than others, and therefore start off more positively charged than other cells. These slightly depolarized cells reach action potential threshold and fire sooner than other cells. The fast-firing cells pass their excitation easily to other cells through the connections between each cell. The fastest depolarizing cells of the heart control the rate, or pace, of contraction. The cells that normally depolarize fastest make up the SA node. The SA node, located in the wall of the right atrium, is the primary pacemaker of the heart.
Spread of Electrical Depolarization through the Myocardium
From the SA node, depolarization spreads rapidly through both atria and soon reaches the AV node, which is located in the lower right atrium near the juncture of the atria and ventricles. When the depolarization reaches the AV node, the electrical signal is delayed shortly before it is passed to the ventricles. During this delay, the atria reach action potential threshold and begin to contract, pumping their last bit of blood into the ventricles. While contraction of the atria is occurring, the electrical depolarization spreads through the AV node down specialized muscle fibers to an area of the ventricles called the bundle of His. Depolarization then spreads rapidly through the left and right bundle branches of the interventricular septum, and from there, down an extensive, wide-reaching group of specialized conducting fibers called Purkinje fibers. In a normal heart, the apex of the ventricle depolarizes first, after which the wave
of depolarization spreads up toward the atria. This wave allows for the efficient ejection of blood from the ventricle.
of depolarization spreads up toward the atria. This wave allows for the efficient ejection of blood from the ventricle.
THE CARDIAC CYCLE
Until the ventricles contract, the AV valves are open and blood flows from the atria into the relaxed, low-pressure ventricles. The aortic and pulmonary valves are closed because pressures are greater in the aortic and pulmonary arteries than in the relaxed ventricles. This allows blood to accumulate in the ventricles. This period of ventricular relaxation is called diastole. Blood volume in the ventricle immediately before ventricular contraction is called end-diastolic volume. When the ventricles contract, pressure inside the ventricles becomes greater than in the atria, and the AV valves snap shut. For a brief period of time, pressure in the aorta and pulmonary arteries is still higher than in the ventricles, so the aortic and pulmonary valves remain closed. With increasing pressure in the ventricles, the aortic and pulmonary valves burst open and blood flows out of the ventricles at high speed and pressure. This period of ventricular contraction is called systole.
With the end of systole, the ventricles relax again. As pressure in the relaxing ventricles falls below that in the aortic and pulmonary arteries, the aortic and pulmonary valves snap shut. Blood entering the atria from the venae cavae and pulmonary veins causes pressure to rebuild in the atria, opening the AV valves. The cycle of filling and emptying begins again.
ARTERIAL PRESSURES
The pulmonary artery and aorta are muscular vessels that expand with the surge of blood they receive from the ventricles. They hold this blood before releasing it into the rest of the vascular system, not in big pulses followed by ebbs of flow, but in a steady stream. Pressure generated in the arteries at the peak of ventricular contraction is much greater than pressure in the arteries when the ventricles are relaxed. Both of these pressures are frequently measured. Systolic pressure is the arterial blood pressure generated during ventricular contraction. Diastolic pressure is the arterial blood pressure generated when the ventricles are relaxed.
HEART SOUNDS
Heart sounds are produced when the AV valves (mitral and tricuspid valves) and the pulmonary and aortic valves snap shut. At least two (and sometimes four) heart sounds can be heard.
The first heart sound (S1) is heard when the AV valves snap shut during ventricular contraction. This sound is somewhat prolonged and low in pitch,
and occurs with the onset of systole when pressure in the ventricles becomes greater than that in the atria. The second heart sound (S2) is shorter and occurs when outlet valves from the ventricles, the pulmonary and aortic valves, snap shut. This happens during diastole, when the ventricles relax and pressures in the pulmonary artery and aorta—which have just received the surging blood—are greater than pressures in the right and left ventricles. Third (S3) and fourth heart sounds (S4) are sometimes heard and are related to the sound of blood reverberating in the ventricles during rapid filling (S3) or entering a ventricle that is stiff (S4), for example, in conditions such as ventricular hypertrophy. S3 heart sounds are considered to be benign in children and young adults; however, after about age 35, it may indicate systolic dysfunction and should be thoroughly assessed.
and occurs with the onset of systole when pressure in the ventricles becomes greater than that in the atria. The second heart sound (S2) is shorter and occurs when outlet valves from the ventricles, the pulmonary and aortic valves, snap shut. This happens during diastole, when the ventricles relax and pressures in the pulmonary artery and aorta—which have just received the surging blood—are greater than pressures in the right and left ventricles. Third (S3) and fourth heart sounds (S4) are sometimes heard and are related to the sound of blood reverberating in the ventricles during rapid filling (S3) or entering a ventricle that is stiff (S4), for example, in conditions such as ventricular hypertrophy. S3 heart sounds are considered to be benign in children and young adults; however, after about age 35, it may indicate systolic dysfunction and should be thoroughly assessed.
CARDIAC OUTPUT
Repeated contractions of the myocardium are the heartbeats. Each beat pumps blood out of the heart. The amount of blood pumped per beat is the stroke volume. Cardiac output (CO), the volume of blood pumped per minute, depends on the product of the heart rate (HR; in beats per minute) and the stroke volume (SV; in milliliters of blood pumped per beat) as shown in the following equation:

Cardiac output of an adult male ranges from 4.5 to 8 L/min. Increased cardiac output is possible with increased heart rate or stroke volume.
The cardiac index is often calculated clinically and offers input on heart performance in an individual. The cardiac index is found by dividing the measured cardiac output by the body surface area of the individual.
Cardiac output can increase or decrease as a result of forces acting intrinsically or extrinsically to the heart; that is, with or without external input. Intrinsic control of cardiac output is determined by the length of the cardiac muscle fibers. Extrinsic control refers to the effect of neural stimulation on the heart.
Intrinsic Control of Cardiac Output
The length of cardiac muscle fibers affects the tension they can produce because of the anatomic arrangement of muscle contractile proteins. In the resting heart, the muscle fibers are stretched to a degree less than that required to produce maximum tension. When cardiac muscle fibers are stretched, more myosin cross-bridges can reach their actin-binding sites, causing an increase in cross-bridge swinging and an increase in cardiac tension and cardiac contractility. This results in an increase in stroke volume and cardiac output (Equation 13-1). Increased stretch of the myofibrils occurs when there is increased filling of the heart; therefore, the tension that is produced by the heart is
proportional to the volume of blood in the heart immediately before ventricular contraction: the end-diastolic volume (also referred to as preload). Because of this response, the heart has reserve capacity to pump more forcefully when the volume of blood flow is increased—for example, with exercise and volume loading.
proportional to the volume of blood in the heart immediately before ventricular contraction: the end-diastolic volume (also referred to as preload). Because of this response, the heart has reserve capacity to pump more forcefully when the volume of blood flow is increased—for example, with exercise and volume loading.
Because an increase in venous return will increase end-diastolic volume, the length-tension relationship of the heart ensures that under most conditions, increased blood flow into the heart will be matched by increased blood pumped out. This serves to return the end-diastolic volume back toward normal, making this response typically of short duration. This intrinsic response of the heart to its own muscle fiber stretch is called Starling’s law of the heart, after Frank Starling, the physiologist who first described it. The length-tension relationship of a normal heart under nonstimulated control conditions is shown in the lower curve of Figure 13-4. Note that, unlike in the skeletal muscle length-tension curve, the normal heart does not fall off the curve at higher fiber length.
The words “under most conditions” in the preceding paragraph refer to the fact that in a damaged heart, overstretch of the ventricle will not improve contractility, and the heart will not be able to pump out the extra blood. Therefore, a damaged heart continues to overfill and eventually becomes overstretched. This situation is characteristic of heart failure, which is described later.
A second reason why the stretch of cardiac muscle fibers determines cardiac output is that with increased venous return, the wall of the right atrium is stretched. This stretch causes an increased firing rate of the SA node and an increased heart rate of up to 20%. This increase in heart rate, coupled with an
increase in stroke volume as a result of extra filling, can dramatically increase cardiac output. However, as mentioned earlier, because an increase in end-diastolic volume increases stroke volume, the intrinsic response to excess volume is usually temporary.
increase in stroke volume as a result of extra filling, can dramatically increase cardiac output. However, as mentioned earlier, because an increase in end-diastolic volume increases stroke volume, the intrinsic response to excess volume is usually temporary.
Extrinsic Control of Cardiac Output
Heart rate and stroke volume are affected by the sympathetic and parasympathetic nervous systems and by circulating hormones.
Sympathetic nerves travel in the thoracic spinal nerve tracts to the SA node and release the neurotransmitter norepinephrine. Norepinephrine binds to specific receptors called β1 adrenergic receptors, present on the cells of the SA node. When this happens, activation of a second messenger system causes increased firing rate of the node, leading to an increase in heart rate. The heart rate is decreased if the activation of the sympathetic nerves and the release of norepinephrine are reduced. An increase or decrease in the heart rate is called a positive or negative chronotropic effect.
Sympathetic nerves also innervate cells throughout the myocardium, causing an increase in the force of each contraction (i.e., the contractility) at any given muscle fiber length. This causes an increase in stroke volume and is called a positive inotropic effect, as shown by the upper curve in Figure 13-4.
Parasympathetic nerves travel to the SA node and throughout the heart through the vagus nerve. Parasympathetic nerves release the neurotransmitter acetylcholine, which slows the rate of depolarization of the SA node and leads to a decrease in heart rate—a negative chronotropic effect. Parasympathetic stimulation to other sites in the myocardium appears to reduce contractility and therefore stroke volume, producing a negative inotropic effect.
Hormonal control of cardiac output mainly involves the adrenal medulla, an extension of the sympathetic nervous system. With sympathetic stimulation, the adrenal medulla releases norepinephrine and epinephrine into the circulation. These hormones travel to the heart and produce positive chronotropic and inotropic responses.
ARTERIES AND VEINS
All blood vessels except the capillaries are composed of three layers: the tunica adventitia, the tunica media, and the tunica intima.
The tunica adventitia is the outermost layer of the blood vessel, away from the lumen of the tube. It is primarily connective tissue and provides the vessels with physical support.
The tunica media is the middle layer of the vessel and is composed of vascular smooth muscle. This layer always has some basal tone, or tension, which can be increased or decreased. An increase in tension of the tunica media results in constriction of the vessel and a narrowing of its lumen, leading to an
increase in the resistance to blood flow through the vessel. Relaxation of the smooth muscle causes dilation of the vessel and decreased resistance to flow. Increases or decreases in the radius of the vessels occur through neural, hormonal, and local mediators of blood flow. Because of their capacity to change their resistance through contraction or relaxation of the smooth muscle, the arterioles in particular are called the resistance vessels of the circulatory system.
increase in the resistance to blood flow through the vessel. Relaxation of the smooth muscle causes dilation of the vessel and decreased resistance to flow. Increases or decreases in the radius of the vessels occur through neural, hormonal, and local mediators of blood flow. Because of their capacity to change their resistance through contraction or relaxation of the smooth muscle, the arterioles in particular are called the resistance vessels of the circulatory system.
The third layer of the blood vessels is the tunica intima, the innermost layer. This single-cell layer is made up of endothelial cells and is surrounded by a basement membrane.
SPECIAL CHARACTERISTICS OF VEINS
The veins, although composed of the tunicae adventitia, media, and intima, have much less smooth muscle than the arteries and arterioles. They are thin vessels that can easily expand to accommodate large volumes of blood and are easily collapsed. Because of their capacity to hold large volumes of blood, the veins are called the capacitance vessels of the circulatory system. This reservoir of venous blood can be called on in times of need when blood volume or pressure is low.
One-way valves are located periodically in the veins. These one-way valves allow blood to proceed toward the heart, but not back the other way. Blood is returned to the heart through the veins as a result of the pressure gradient that exists between the veins and the heart. Surrounding skeletal muscles contribute to returning venous blood to the heart by contracting and thus squeezing the veins. The valves prevent the blood squeezed up toward the heart from falling back down when the muscles relax. If one stands for a long period of time, the muscles relax and the valves do not close entirely, allowing blood to pool in the feet and ankles.
CAPILLARIES
The smallest of the blood vessels are the capillaries, with a diameter between 4 and 9 μm, barely large enough for a red blood cell to flow through. The capillaries are composed only of endothelial cells. Lipid-soluble substances, such as oxygen and carbon dioxide, pass out of the capillaries into the interstitial space by diffusing across the endothelial cells. Substances that are not lipid soluble, such as small ions and glucose, may move between the endothelial cells through intercellular clefts or pores to reach the interstitial space. Because the diameter of the capillary pores is much smaller than the diameter of the plasma proteins and red blood cells, and because neither is lipid soluble, proteins and red cells are prohibited from moving out of the vascular system into the interstitial space.
Precapillary Sphincter
Immediately proximal to the capillaries are the meta-arterioles, which deliver blood to the capillaries through a precapillary sphincter. The precapillary sphincter is a smooth muscle fiber encircling the entrance to the capillary. This fiber is not innervated by nerves, but responds to hormonal and local mediators of blood flow.
Bulk Flow Across the Capillary
Bulk flow is the movement of a fluid as a result of a pressure gradient from high to low. In the vascular system, fluid moves back and forth between the capillaries and the interstitial fluid. Interstitial fluid is a plasmalike filtrate surrounding all cells. It can store fluid in times of high plasma volume, or resupply the vascular system in times of plasma loss.
There are four forces affecting the bulk flow of fluid across a capillary into the interstitial space. They include capillary pressure, interstitial hydrostatic pressure, plasma colloid osmotic pressure, and interstitial fluid colloid osmotic pressure.
Capillary hydrostatic pressure is the remainder of the mean arterial pressure generated by the heart. For most capillaries, this pressure averages approximately 18 mm Hg over the length of the capillary (Fig. 13-5), with pressure at the arteriolar end (28 mm Hg) significantly greater than the pressure at the venous end (10 mm Hg). Capillary pressure is a reflection of mean blood pressure.
Therefore, if blood pressure increases, capillary pressure increases. If blood pressure decreases, capillary pressure decreases. Capillary pressure favors filtration of plasma out of the capillary into the interstitial space.
Therefore, if blood pressure increases, capillary pressure increases. If blood pressure decreases, capillary pressure decreases. Capillary pressure favors filtration of plasma out of the capillary into the interstitial space.
Interstitial hydrostatic pressure is the pressure exerted by fluid in the interstitial space. Interstitial hydrostatic pressure is primarily caused by water. If positive, it opposes filtration of plasma out of the capillary; if negative, it draws fluid out of the capillary. Recent research suggests that the pressure is negative in most tissues, averaging approximately 3 mm Hg. In some tissues, it may be positive and oppose filtration.
Plasma (capillary) colloid osmotic pressure refers to osmotic pressure exerted by plasma proteins. Plasma colloid pressure opposes filtration of plasma out of the capillary. This pressure develops when water is pushed out of the capillary by the hydrostatic pressure and the proteins are too large and too charged to follow. The concentration of protein left behind increases, causing an increase in osmotic pressure. This pressure serves to draw water back into the capillary. Plasma colloid osmotic pressure in most capillaries averages approximately 28 mm Hg.
Interstitial fluid colloid osmotic pressure is normally a small force. Few proteins escape across the capillary into the interstitial compartment. Those that do are rapidly taken up into vessels of the lymph system. The lymph vessels return the proteins to the bloodstream by delivering them into the vena cava and the right atrium. Interstitial fluid colloid osmotic pressure averages approximately 8 mm Hg.
Adding up the forces, filtration (18 + 3 + 8 mm Hg = 29 mm Hg) nearly balances reabsorption (28 mm Hg) and little net movement of fluid across the capillary occurs (see Fig. 13-5). Extra fluid in the interstitial space is reabsorbed by the lymph flow.
BLOOD FLOW
Blood travels in the vascular system by bulk flow, the movement of a fluid through a tube based on the pressure difference between one end of the tube and the other. The pressure of the blood as it leaves the heart (P1) minus the pressure in a downstream vessel (P2), divided by the resistance offered by the blood vessels (R), determines the blood flow (F) through the vascular system, as expressed in the following equation:

Blood Pressure
Pressure at the beginning of the aorta is generated by the left ventricle. This pressure varies between approximately 120 mm Hg during systole and 80 mm Hg during diastole. Because diastole lasts longer than systole, the average, or mean, blood pressure equals approximately 40% of systolic pressure plus 60%
of diastolic pressure. The systolic pressure represents the maximum pressure on the aorta and major vessels and the diastolic pressure reflects the minimum pressure on the arteries. The difference in the systolic and diastolic pressure is referred to as pulse pressure.
of diastolic pressure. The systolic pressure represents the maximum pressure on the aorta and major vessels and the diastolic pressure reflects the minimum pressure on the arteries. The difference in the systolic and diastolic pressure is referred to as pulse pressure.
As blood moves through the large and small arteries, some pressure is lost. Much more is lost as blood traverses the arterioles and capillaries. By the time blood flow reaches the capillary, blood pressure at the arteriole end of the capillary has decreased to approximately 35 mm Hg for most capillary beds. With movement through the capillary, this pressure decreases to 10 mm Hg at the venous end, resulting in a mean blood pressure in the capillary of approximately 18 mm Hg. By the time the blood reaches the vena cava, the pressure is zero.
Thus, the pressure gradient affecting flow is large between the aorta and the vena cava (90 to 0 mm Hg). This is the force that drives the blood through the systemic circulation.
Resistance
Resistance to flow through a vessel depends on the length and radius of the vessel, and on the viscosity of the fluid. In the body, the length of the blood vessels is essentially fixed. Although potentially variable, blood viscosity is also fixed. Therefore, when discussing resistance to blood flow in the vascular system, one usually considers only the radius of the blood vessels. Because of the dynamics of flow through a tube, a small decrease in the radius causes an enormous increase in resistance to flow. This is true both for blood flowing through a blood vessel and for water flowing through a hose or a pipe.
The smaller the vessel, the greater the effect narrowing that vessel has on blood flow. Varying the radius of the large arteries does not significantly affect blood flow. Likewise, because veins are so distensible, they offer little resistance to flow. Instead, resistance to blood flow is determined by the radius of the arterioles, thus making the arterioles the resistance vessels of the cardiovascular system.
Narrowing an arteriole decreases blood flow downstream into the capillaries and veins fed by that arteriole, backing up the blood upstream. Because blood pressure depends on blood flow, narrowing the arterioles decreases blood pressure downstream and increases blood pressure upstream.
In contrast, if the arterioles are dilated, flow increases, resulting in increased downstream pressure and decreased pressure upstream. With the dilation, force is decreased. Control of arteriole diameter is an intricate balance between local effects and nervous and hormonal stimulation.
Capillary Resistance to Blood Flow
The capillaries offer a great deal of resistance to blood flow because they are so narrow. However, because they have no smooth muscle, their diameter
cannot be varied, so changes in capillary diameter cannot cause an increase or decrease in blood flow. The meta-arterioles immediately preceding the capillaries do change in diameter and affect capillary blood flow. Because of the extensive surface area covered by all the capillaries, blood flow through them is slow, allowing ample time for the diffusion of oxygen and carbon dioxide to occur.
cannot be varied, so changes in capillary diameter cannot cause an increase or decrease in blood flow. The meta-arterioles immediately preceding the capillaries do change in diameter and affect capillary blood flow. Because of the extensive surface area covered by all the capillaries, blood flow through them is slow, allowing ample time for the diffusion of oxygen and carbon dioxide to occur.
Total Peripheral Resistance
Resistance in the systemic vascular system is referred to as total peripheral resistance (TPR). It is impossible to measure resistance directly. Resistance in the cardiovascular system is calculated by measuring flow and pressure. The resistance equals pressure divided by flow. Resistance to flow in the pulmonary vascular system is much less than in the systemic system. The amount of force that the ventricle must generate to overcome vascular resistance is referred to as afterload.
CONTROL OF MEAN ARTERIAL BLOOD PRESSURE
From the previous discussion, it should be apparent that in the vascular system it is difficult to discuss blood flow without referring to blood pressure. The variable regulated by the body, and usually measured clinically, is the systemic arterial blood pressure (BP). Equation 13-3 is used to describe the variables controlling systemic mean arterial blood pressure:

where, for the cardiovascular system,
BP is the mean arterial blood pressure,
CO is the cardiac output (which equals HR × SV). Note: CO replaces F from Equation 13-2.
TPR is the total peripheral resistance.
Blood pressure control depends on sensors that continually measure blood pressure and send the information to the brain. The brain integrates all incoming information and responds by sending efferent (outgoing) stimulation to the heart and vasculature through the autonomic nerves. Various hormones and locally released chemical mediators add to the control of blood pressure.
Sensors
Blood pressure is continually monitored by sensors called baroreceptors (pressure receptors). There are baroreceptors in the carotid artery (in the neck) and in the aortic arch where the aorta leaves the heart; these sensors are called the carotid and aortic baroreceptors, respectively. There are baroreceptors located
in the arterioles supplying the kidney nephrons. Receptors in both atria and in the pulmonary artery also respond to changes in pressure. Because the atrial and pulmonary artery receptors are in low-pressure areas of the vasculature, they are called low-pressure receptors.
in the arterioles supplying the kidney nephrons. Receptors in both atria and in the pulmonary artery also respond to changes in pressure. Because the atrial and pulmonary artery receptors are in low-pressure areas of the vasculature, they are called low-pressure receptors.
All baroreceptors act as stretch receptors that respond to changes in blood pressure. Their stretch increases with increased blood pressure. This stretch increase causes afferent neurons receiving information from the receptors to increase their rate of firing. These neurons travel to the brain and innervate its cardiovascular center. A decrease in blood pressure decreases the stretch of the baroreceptors, which reduces the firing of the afferent nerves innervating the cardiovascular center.
Chemoreceptors located in the carotid bodies and the aorta, respond to changes in oxygen, carbon dioxide, and hydrogen ion concentration in arterial blood. Low blood pressure, decreased oxygen, or accumulation of metabolic end products stimulates the sympathetic nervous system causing the chemoreceptors and baroreceptors to respond and signal the brain. When blood pressure drops, the parasympathetic nervous system is inhibited and the sympathetic system is stimulated to increase heart rate. Chemoreceptors cause vasoconstriction and play a vital role in ventilation (see Chapter 14).
Integrating Center for the Control of Blood Pressure
The cardiovascular center in the brain is part of the reticular formation and is located in the lower medulla and pons. This center is the site of neural control for blood pressure regulation. If a change in blood pressure has occurred, the cardiovascular center activates the autonomic nervous system, leading to changes in sympathetic and parasympathetic stimulation to the heart and sympathetic stimulation to the entire vascular system. Resistance of the vasculature is altered and blood flow and blood pressure are affected.
Efferent Neural Innervation of the Vascular System
Sympathetic nerves stimulate heart rate and contractility by binding to β1 receptors in the heart. Parasympathetic nerves decrease heart rate by binding to cholinergic receptors. In addition, sympathetic nerves traveling in the thoracic and upper lumbar spinal tracts influence blood pressure by exerting control over virtually the entire peripheral vascular system (except the capillaries) through innervation of the tunica media (the smooth muscle).
At most blood vessels, sympathetic nerves release norepinephrine, which binds to specific receptors on the smooth muscle cells, called alpha (α) receptors. Stimulation of the α receptors causes the smooth muscle to contract, constricting the vessel, which increases TPR and therefore increases blood pressure.
Blood vessels supplying skeletal muscle have a different type of receptor, called beta2 (β2) receptors, which, when stimulated by norepinephrine, cause the
vessels to relax. It appears that this sympathetic vasodilatory response plays a significant role only in the anticipatory response to exercise, perhaps serving to prime the skeletal muscle with oxygen and nutrient support before exercise onset.
vessels to relax. It appears that this sympathetic vasodilatory response plays a significant role only in the anticipatory response to exercise, perhaps serving to prime the skeletal muscle with oxygen and nutrient support before exercise onset.
Skeletal muscle blood vessels also possess receptors for acetylcholine. These receptors are called muscarinic receptors and do not appear to be innervated by parasympathetic neurons. However, they respond to acetylcholine released by certain sympathetic cholinergic neurons. These neurons also supply the vascular smooth muscle in skeletal muscle and cause relaxation of the vessels, thus increasing blood flow through these vessels.
Hormonal Control of the Vascular System
There are several hormones that control the resistance of the vascular system. These hormones are released directly in response to changes in blood pressure, in response to neural stimulation, or both.
Norepinephrine and Epinephrine
The catecholamines, norepinephrine and epinephrine, are released from the adrenal medulla in response to activation of the sympathetic nervous system. Both substances act like norepinephrine released from nerve terminals and bind to á receptors to cause vasoconstriction, or to β2 receptors to cause vasodilation of arterioles supplying skeletal muscles. Norepinephrine and epinephrine from the adrenal medulla also bind to β1 receptors and increase heart rate.
Renin-Angiotensin System
Renin is produced and stored by the kidney. When renal baroreceptors sense a change in blood pressure the release of renin is increased or decreased. Renin release is also stimulated by sympathetic nerves to the kidney. If blood pressure is high, release of the hormone renin is decreased. When blood pressure drops, renin is released and acts as an enzyme to convert the protein angiotensinogen to angiotensin I.
Angiotensin I is a 10-amino acid protein, which is immediately split by angiotensin-converting enzyme (ACE) into the 8-amino acid peptide, angiotensin II. ACE is present in the blood vessels of the lungs and is the same enzyme that breaks down (and inactivates) the vasodilator hormone bradykinin. Blocking the action of ACE blocks the production of angiotensin II and the breakdown of bradykinin.
Angiotensin II is a powerful vasoconstrictor that primarily causes constriction of the small arterioles. This causes an increase in resistance to blood flow and an increase in blood pressure. The increase in blood pressure then acts in a negative feedback manner to reduce the stimulus for further renin release. Angiotensin II also circulates to the adrenal gland and causes cells of the adrenal cortex to synthesize another hormone, aldosterone.
Aldosterone.
Aldosterone circulates to the kidney and causes cells of the distal tubule to increase sodium reabsorption. Under many circumstances, reabsorption of water follows that of sodium, leading to an increase in plasma volume. An increase in plasma volume increases stroke volume, and hence cardiac output. It also causes increased blood pressure.
Renin-Angiotensin-Aldosterone Feedback Cycle.
It should be emphasized that the stimuli causing the release of renin—decreased blood pressure and decreased plasma sodium concentration—are reversed by the actions of angiotensin II and aldosterone. This is an excellent example of a negative feedback cycle.
Antidiuretic Hormone.
Antidiuretic hormone (ADH), also called vasopressin, is released from the posterior pituitary in response to increased plasma osmolality (decreased water concentration) or decreased blood pressure.
ADH is a potent vasoconstrictor with the potential to increase blood pressure by increasing the resistance to blood flow. Under most circumstances, except perhaps during severe hemorrhage, levels of circulating ADH are too low to affect the arterioles. However, ADH controls the reabsorption of water across the collecting ducts of the kidney back into the bloodstream. This effect influences blood pressure by increasing plasma volume and therefore cardiac output. Without ADH, water does not follow sodium reabsorption in the kidney and severe dehydration may occur. Reabsorption of water in response to ADH reduces the stimuli (increased plasma osmolality and decreased blood pressure) for ADH release.
Atrial Natriuretic Peptide.
Atrial natriuretic peptide (ANP) is a hormone released from cells of the right atrium in response to an increase in blood volume. ANP acts on the kidney to increase the excretion of sodium ion (natriuresis). Because water will follow sodium in the urine, ANP serves to decrease blood volume and blood pressure.
Summary of Blood Pressure Control
With a decrease in blood pressure, baroreceptor information is transmitted to the cardiovascular center in the brain. This causes the stimulation of sympathetic output to the heart and vascular system, increasing heart rate and TPR. Parasympathetic output is decreased, also increasing heart rate. Renin release increases, causing increased angiotensin II, which directly increases TPR and aldosterone synthesis. Increased aldosterone increases sodium reabsorption and, in the presence of ADH, water reabsorption. Increased plasma volume, stroke volume, and cardiac output result. Capillary pressure decreases directly with blood pressure and indirectly from sympathetic constriction of the arteriole
feeding the capillary. This serves to decrease filtration of fluid out of the capillary. All of these responses serve to increase blood pressure toward normal, by increasing heart rate, stroke volume, and TPR (Fig. 13-6).
feeding the capillary. This serves to decrease filtration of fluid out of the capillary. All of these responses serve to increase blood pressure toward normal, by increasing heart rate, stroke volume, and TPR (Fig. 13-6).
In contrast, if blood pressure increases, baroreceptor responses cause a decrease in sympathetic stimulation to the heart and vascular smooth muscle, and heart rate and TPR decrease. Increased parasympathetic stimulation to the heart contributes to the decrease in heart rate. There is a decrease in renin and ADH release, reducing TPR and plasma volume. ANP release increases. All these responses serve to decrease blood pressure toward normal.
Although hormonal and neural mechanisms can quickly regulate blood pressure, the effect is short-term. The kidneys are responsible for long-term blood pressure control by regulating extracellular fluid volume (ECF). For example, in response to ECF from increased water and sodium retention, the kidney responds by increasing the excretion of water and sodium. Autoregulation of blood flow also impacts how fluid volume affects blood pressure.
AUTOREGULATION OF BLOOD FLOW
In general, blood pressure is controlled through neural and hormonal influences. However, individual tissues have mechanisms that regulate their own blood flow. This is accomplished by local vasodilation or vasoconstriction of meta-arterioles and precapillary sphincters. This local control of blood flow is
called autoregulation, and is the means by which some organs can maintain constant blood flow over a wide range of blood pressure, from approximately 70 to 180 mm Hg.
called autoregulation, and is the means by which some organs can maintain constant blood flow over a wide range of blood pressure, from approximately 70 to 180 mm Hg.
Theories of Autoregulation
Several theories are proposed to explain local control of blood flow. The most widely accepted is that chemical mediators are released by metabolizing cells that bind to meta-arterioles or precapillary sphincters, causing them to open or shut to blood flow.
Chemical mediators that control local blood flow include adenosine (a metabolite of ATP), carbon dioxide, histamine, lactic acid, potassium ions, and hydrogen ions. All of these substances except histamine are by-products of metabolism; as cell metabolism increases, so do their concentrations. This serves to match increased metabolic activity with increased blood flow. Mast cells present throughout the interstitial space release histamine in response to immune stimulation or local injury. Adenosine appears to particularly regulate local blood flow in the heart.
An alternative theory concerning local control suggests that the meta-arterioles and capillary sphincters sense an oxygen or nutrient deficit that causes them to relax, thereby increasing blood flow to the surrounding cells.
Other Chemical Mediators Influencing Blood Flow
Various other chemicals are released by the blood vessels or by mediators of inflammation or healing, which affect blood flow to an area.
Nitric Oxide
Endothelial cells of the small arteries and arterioles respond to the binding of various vasoactive substances such as acetylcholine with the production of the vasodilator nitric oxide (previously called endothelial-derived relaxing factor). Nitric oxide diffuses through endothelial cells to underlying smooth muscle cells, causing endothelial-dependent relaxation of the smooth muscle and inhibition of platelet aggregation. Nitric oxide release also occurs with increased blood flow through a vessel, allowing local dilation of the microvasculature to be matched by dilation of the small arteries and arterioles. Drugs that boost nitric oxide synthesis or prevent its breakdown are used to improve blood flow in a variety of clinical situations.
Endothelin
Endothelial cells also release endothelin, a 21-amino acid peptide that acts as a potent constrictor of vascular smooth muscle. Endothelin release is stimulated by angiotensin II, ADH, thrombin, cytokines, reactive oxygen species, and shearing forces acting on the vascular endothelium. Its release is inhibited by prostacyclin and nitric oxide. Deleterious effects of overproduction of
endothelin on vascular smooth muscle include prolonged vasoconstriction, vascular hypertrophy, cell proliferation, and fibrosis. In addition, by binding to receptors on endothelial cells that are different from the ones on vascular smooth muscle, endothelin increases capillary permeability and contributes to inflammation. Endothelin has a long half-life (length of presence in the circulation or interstitial fluid), so even small changes in its production or clearance have significant impact on the vascular system. Overproduction of endothelin is implicated in numerous pathologies, including pulmonary hypertension, myocardial hypertrophy, and heart failure. Drugs to block endothelin receptors are being tested in a variety of clinical situations.
endothelin on vascular smooth muscle include prolonged vasoconstriction, vascular hypertrophy, cell proliferation, and fibrosis. In addition, by binding to receptors on endothelial cells that are different from the ones on vascular smooth muscle, endothelin increases capillary permeability and contributes to inflammation. Endothelin has a long half-life (length of presence in the circulation or interstitial fluid), so even small changes in its production or clearance have significant impact on the vascular system. Overproduction of endothelin is implicated in numerous pathologies, including pulmonary hypertension, myocardial hypertrophy, and heart failure. Drugs to block endothelin receptors are being tested in a variety of clinical situations.
Serotonin
Serotonin (5-hydroxytryptamine) is primarily released by platelets drawn to an area of injury or inflammation. Effects of serotonin may be vasodilatory or vasoconstricting, depending on the site of release. Serotonin’s ability to vasoconstrict and decrease blood flow appears to be one mechanism whereby platelets control or reduce bleeding.
Bradykinin
Bradykinin, like all members of the kinin family, is a small polypeptide that acts as a potent vasodilator of arterioles and as a mediator to increase capillary permeability. Bradykinin is produced in the plasma or interstitial fluid by enzymatic splitting of a serum globulin in response to vascular or tissue injury or inflammation. The half-life of bradykinin is short. Normally, it is broken down rapidly by circulating ACE or another enzyme, carboxypeptidase.
The effects of bradykinin are increased local blood flow, increased capillary permeability, and decreased vascular resistance. These effects allow delivery of mediators of the inflammatory and immune systems to a site of injury. Blockage of ACE by various pharmaceutical agents prolongs the half-life of bradykinin and its effects.
Prostaglandins
There are many different types of prostaglandins. Some cause dilation of the vascular system and some cause constriction. Prostaglandins are derived from the metabolism of arachidonic acid by cyclooxygenase (COX) enzymes one (COX1) and two (COX2). Arachidonic acid is present in all cell membranes and is released with tissue injury. Prostaglandins work to control local blood flow. They may circulate to affect distant cells.
One main group of prostaglandins, those of the E series, cause local vasodilation and increased blood flow, which makes them important mediators of inflammation. Prostaglandins of the I series, especially PGI2, called prostacyclin, also are vasodilatory. PGI2 inhibits platelet aggregation and blood clotting. Thromboxane A2 is an important prostaglandin that causes vasoconstriction and blood clotting.
Prostaglandin synthesis is inhibited by drugs that block the function of the COX enzymes, including aspirin and nonsteroidal anti-inflammatory drugs (NSAIDs), and by specific COX enzyme inhibitors. Low concentration of aspirin particularly appears to cause a long-lasting block in production of thromboxane A2. Glucocorticoids, including endogenously released cortisol and dexamethasome provided therapeutically, block prostaglandin synthesis at an early stage after membrane injury, prior to the formation of arachidonic acid, and are potent anti-inflammatory agents.
THE LYMPH SYSTEM
The lymph system consists of closed-end vessels that course through almost the entire interstitial fluid space. Lymph fluid is derived from interstitial fluid and is therefore very similar in composition to plasma.
Lymph Flow
The lymph system consists of small capillaries that drain into larger lymph vessels. Like blood vessels, these larger vessels are composed of smooth muscle and endothelial cells. Lymph from the lower body flows up the thoracic duct and empties into the left jugular and subclavian veins. Lymph flow from the left arm, shoulder, and left side of the head travels through the thoracic duct and then into the left jugular and subclavian arteries. Lymph flow from the right arm and right side of the neck and head empties into the right jugular and subclavian veins. The lymphatic system is illustrated in Figure 13-7.
Movement of lymph results from contraction of the smooth muscle lining the lymph vessels in response to its stretch, and the pumping action of the surrounding skeletal muscles. Valves present in lymph vessels prevent backflow.
Role of the Lymph System
The lymph system has three essential roles in the body, all of which depend on the greater permeability of lymph capillaries compared to blood capillaries.
First, lymph capillaries retrieve any proteins that escape from the capillaries into the interstitial fluid. These proteins move easily into lymph vessels and are then returned to the blood circulation via the thoracic duct. This is essential because it allows interstitial colloid osmotic pressure to remain low. If proteins were allowed to accumulate in the interstitial space, interstitial colloid osmotic pressure would increase. This would result in increased forces favoring filtration into the interstitial fluid, which would soon cause massive interstitial edema. Death of the individual could occur from circulatory collapse.
The second essential role played by the lymph system involves the absorption of fats from the small intestine. In the small intestine, fats and fat-soluble vitamins are absorbed into small lymph vessels called lacteals, and are then delivered to the general circulation. Fat and the fat-soluble vitamins are required for life.
![]() FIGURE 13-7 The lymphatic system.(From Anatomical Chart Company [2010]. Atlas of pathophysiology [3rd ed.]. Philadelphia: Lippincott Williams & Wilkins.) |
The third essential role played by the lymph system involves immune function. Because of the high permeability of the lymph capillaries, bacteria and other microorganisms enter the lymph system from infected areas and are transported to and through lymph nodes, where they become trapped and removed from the circulation. Lymph nodes are an intertwining meshwork of vessels filled with tissue macrophages and T and B cells; bringing bacteria and other cellular debris to the lymph nodes allows the immune and inflammatory cells an opportunity to protect the host from widespread infection. Lymph nodes are spaced intermittently along the lymph vessels.
FETAL CIRCULATION
There are several major differences between fetal circulation and the circulation of infants, children, and adults. While in utero, a fetus does not receive oxygen through its own lungs. Rather, maternal oxygen is delivered across the placenta into the umbilical vein. The umbilical vein delivers oxygen-rich blood to the right side of the fetal heart through the vena cava. Because of the maternal source of oxygen, the fetal lungs and most of the blood vessels supplying them are collapsed, causing high resistance to blood flow through the fetal lungs, especially when compared to flow through the fetal systemic circulation, which offers low resistance because of the wide-open vessels of the placenta (Fig. 13.8).
There are also structural differences that characterize fetal circulation. In the fetus, there are two connections (shunts) that exist to take advantage of the maternal oxygen source and the high resistance of the pulmonary circulation. The first of these connections is an opening between the right atrium and the left atrium, called the foramen ovale. Because resistance is so high in the pulmonary circuit leaving the right ventricle, fetal blood travels in the direction of lower resistance: from right atrium to left through the foramen ovale. Because blood entering the vena cava in the fetus has already been oxygenated by passage through the placenta, this right to left shunting is an efficient adaptation. Well-oxygenated blood is delivered to the systemic (left side) circulation without the need to send blood through the collapsed, nonfunctioning pulmonary system.
The second shunting system between the right and left sides of circulation in the fetus is a vascular connection between the pulmonary artery and the aorta. This connection, called the ductus arteriosus, allows oxygenated blood leaving the right side of the heart to bypass the fetal lungs and flow directly into the low resistance of the systemic circulation. It should be noted that the fetal lungs do receive a small amount of blood flow through the pulmonary artery, allowing for their continued growth and development.
NEWBORN CIRCULATION
With birth, the situation changes dramatically and suddenly. The newborn is no longer supplied with oxygen from the placenta because the low-resistance vessels of the placenta are no longer connected to the fetus. At birth, the newborn
separates from the placenta, fluid in the lungs is squeezed out, and the newborn takes a deep breath, opening up the lungs and the blood vessels flowing through them. Immediately at birth, resistance of the pulmonary circulation falls while resistance of the systemic circulation increases. Blood flow no longer shunts right to left through the foramen ovale or the ductus arteriosus because those directions now offer higher resistance to flow. These shunt passages normally begin to close within a few hours after birth.
separates from the placenta, fluid in the lungs is squeezed out, and the newborn takes a deep breath, opening up the lungs and the blood vessels flowing through them. Immediately at birth, resistance of the pulmonary circulation falls while resistance of the systemic circulation increases. Blood flow no longer shunts right to left through the foramen ovale or the ductus arteriosus because those directions now offer higher resistance to flow. These shunt passages normally begin to close within a few hours after birth.
TESTS OF CARDIOVASCULAR FUNCTIONING
The Electrocardiogram
The electrocardiogram (ECG) is the measurement of the electrical currents of the heart. Contraction of the atria and ventricles results from action potentials occurring simultaneously in all muscle cells of the atria, followed by all muscle cells of the ventricles. Electrodes placed in specific locations on the body can
detect these action potential currents. The currents can then be graphically displayed and interpreted.
detect these action potential currents. The currents can then be graphically displayed and interpreted.
Currents Measured by the Electrocardiogram
There are three currents produced in the normal ECG, as shown in Figure 13-9.
The P wave corresponds to atrial depolarization.
The QRS complex (beginning of Q wave to end of S wave) corresponds to depolarization of the ventricles.
The T wave corresponds to repolarization of the ventricles.
Repolarization of the atria occurs during the QRS complex and is nondistinguishable. The U wave is an inconsistent finding that may represent slow repolarization of the papillary muscles involved in opening and closing the AV valves. Normal sinus rhythm is the expected rhythm of the heart, driven by the SA node and passed along the normal, intact conduction system.
Patterns of the Electrocardiogram
Several patterns in the normal ECG stand out. First, the pattern of the three waves repeats itself with each beat. A timescale is usually shown on the recording, allowing one to determine the heart rate by counting any one of the waves over time. The P wave or the QRS complex can be counted.
Second, the QRS complex always follows the P wave in a normal beating pattern because the atria undergo an action potential and contract first. Their action potential subsequently spreads to the ventricles. The time between the end of the P wave and the beginning of the QRS complex reflects the time during which the action potential is delayed at the AV node.
Third, the size of the atrial depolarization, as measured by the height of the P wave, is less than the depolarization of the ventricles, as measured by the height of the QRS complex. This reflects the much greater muscle mass of the ventricles compared to the atria. The ventricular depolarization is a rapid spike, as shown by the narrow displacement of the QRS complex, indicating that conduction throughout the ventricle is rapid, and that the entire ventricle contracts as one quick-firing unit. Increased horizontal spread of the QRS complex occurs with prolonged conduction of the electrical impulse through the ventricles, indicating ventricular hypertrophy. A bizarre QRS complex may indicate cardiac cell death.
Measurement of Cardiac Enzymes
When cardiac muscle cells die during a myocardial infarct (MI), they release their intracellular contents. Specific proteins and enzymes normally present only inside cardiac cells can be measured in the blood. This allows one to accurately diagnose the existence and frequently the extent of myocardial cell death. Because different enzyme levels are elevated at different times after infarction, the timing of the infarct can be determined.
Proteins released after injury to the myocardial cells include myoglobin, normally found only in skeletal and cardiac muscle cells, and the cardiac-specific contractile proteins, troponin T, C, and I. Highly sensitive bedside laboratory kits, capable of measuring the presence of very low amounts of serum troponin T and I within a short time of a suspected infarct, have the capacity to revolutionize early detection of an MI. Because troponin T elevates early and stays elevated for up to two weeks, it is useful for diagnosing cardiac events that may have occurred in the past 14 days. C-reactive protein is also released in the presence of myocardial injury. Myocardial creatine kinase (CK-MB) is an enzyme that is released with cardiac cell death. Lactic acid dehydrogenase (LDH), and serum glutamic oxaloacetic transaminase (SGOT) may also be elevated after myocardial injury, but are no longer considered the best indicators. The plasma concentration of each of these enzymes and proteins varies depending on the time since injury and the extent of the cell damage. Table 13-2 summarizes the most definitive serum markers of myocardial ischemia.
Stress Testing
Cardiac stress testing involves having an individual exercise up to his or her maximal capacity while observers monitor physical symptoms and the ECG. In a simple exercise stress test, the patient is asked to either walk on a treadmill or ride an exercise bike. The pattern of the ECG is observed for alterations in rhythm, the presence of AV blocks, and evidence of ST-segment changes indicative of hypoxia. Onset of physical symptoms, such as chest pain and extreme shortness of breath, is monitored.
TABLE 13-2 Serum Markers of Myocardial Ischemia | ||||||||||||||||||||||||||||
---|---|---|---|---|---|---|---|---|---|---|---|---|---|---|---|---|---|---|---|---|---|---|---|---|---|---|---|---|
|
Nuclear Stress Testing
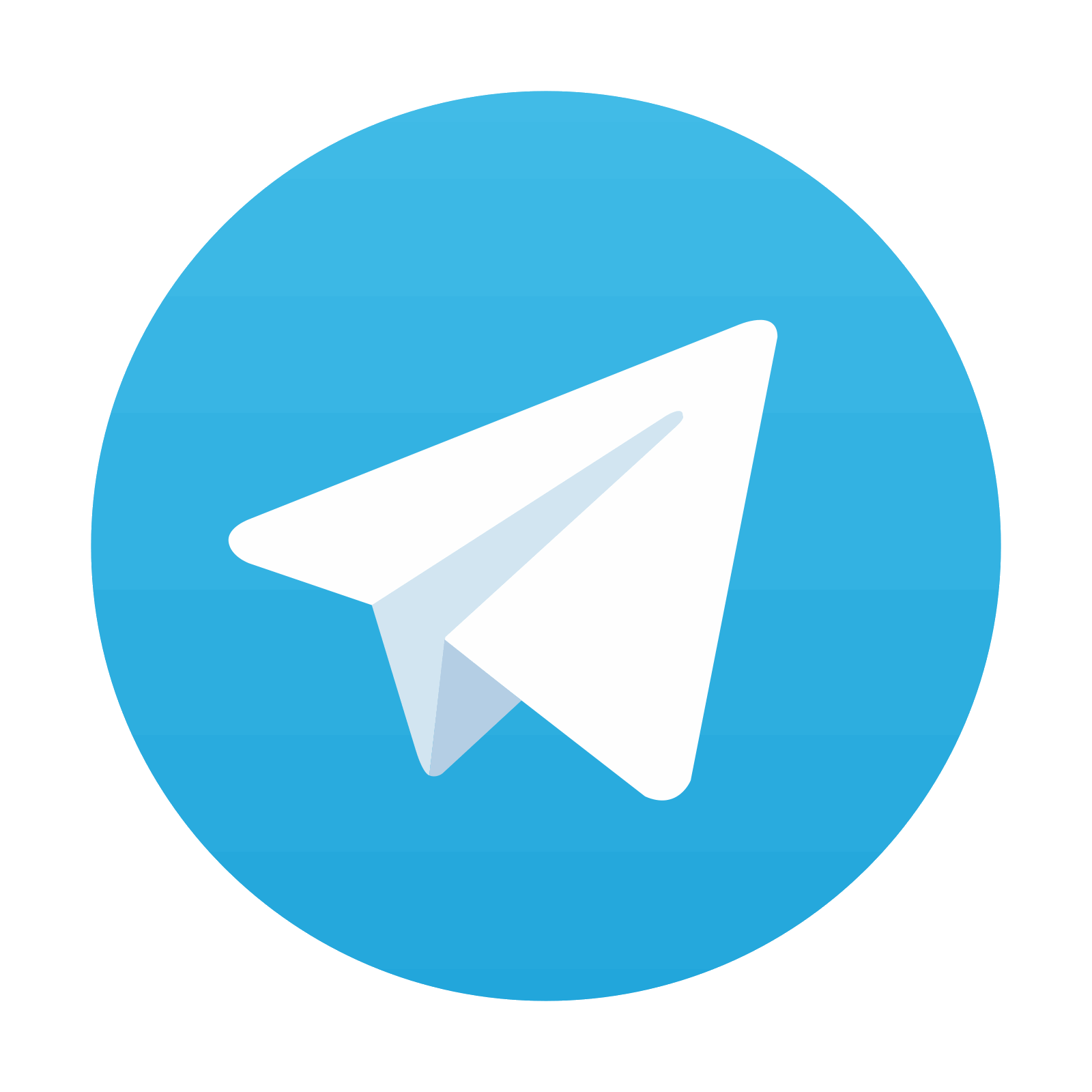
Stay updated, free articles. Join our Telegram channel
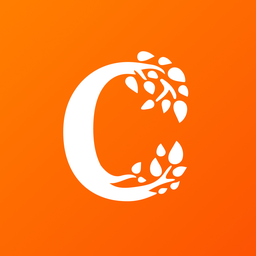
Full access? Get Clinical Tree
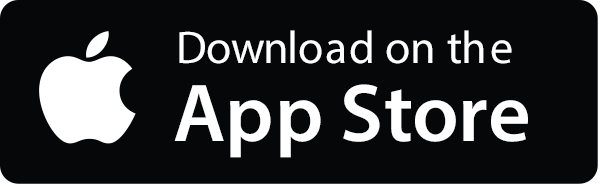
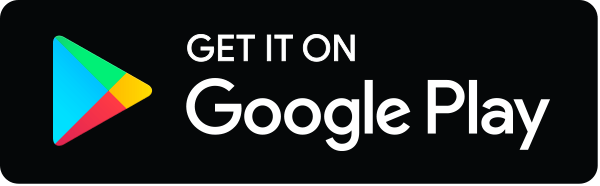
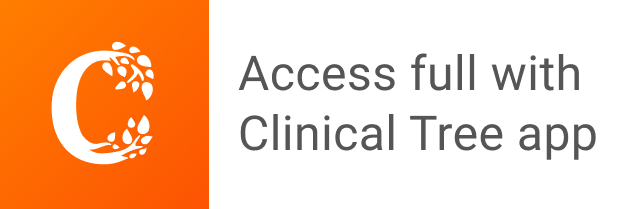