Blood pressure is maintained within fairly narrow limits due to modulation by a series of physiological reflexes that are triggered by both acute and chronic changes in blood pressure. There are both short-term and long-term control mechanisms. Important regulatory systems are:
The autonomic nervous system regulates arterial blood pressure in several ways.



The autonomic nervous system is normally responsible for immediate regulation of blood pressure. Change in systemic blood pressure is detected by baroreceptors (stretch receptors) in the aorta and carotid arteries. When blood pressure rises, stretch of the baroreceptors increases afferent nerve impulses to a coordinating area in the medulla which controls the autonomic outflow to the cardiovascular system. The increase in afferent impulses inhibits sympathetic nervous system output and increases parasympathetic nervous system outflow, returning the blood pressure to normal. The opposite occurs when blood pressure falls (Fig. 6.1).
Fig. 6.1 The role of baroreceptors.
Increased blood pressure increases neural discharge from the baroreceptors (stretch receptors) in the carotid sinus and aortic arch, resulting in a compensatory inhibition in sympathetic outflow from the rostral ventrolateral medulla (RVLM) and an increase in the parasympathetic outflow from the cardioinhibitory vagal nucleus (VN). Both effects act to lower blood pressure, which is also influenced by control from higher centres acting at the nucleus of the tractus solitarius (NTS). See Fig. 6.7 for sites of action of centrally acting antihypertensive drugs. +, stimulation; –, inhibition.
A slower compensatory mechanism for a reduction in blood pressure is initiated by the release of renin from the juxtaglomerular apparatus of the kidney (Fig. 6.2). The major stimuli leading to renin release are reduced renal blood flow (often as a result of a decrease in blood pressure), decreased Na+ in the renal distal tubule and direct sympathetic stimulation via β1-adrenoceptors. Renin is a protease that acts on circulating renin substrate (angiotensinogen) to release the decapeptide angiotensin I. This is further cleaved by angiotensin-converting enzyme the octapeptide angiotensin II. There are several additional enzymatic pathways for generating angiotensin II that do not involve ACE (see Fig. 6.8). Angiotensin II acts on various tissues via specific angiotensin AT1 and AT2 receptors (Fig. 6.2). Its action at the AT1 receptor produces potent direct vasoconstriction, and also enhances sympathetic nervous tone by facilitating presynaptic neuronal release of noradrenaline and through stimulation of central sympathetic outflow. Angiotensin II has a number of additional properties which promote salt and water retention (Fig. 6.3), one of the most powerful being the release of aldosterone from the adrenal cortex. Aldosterone acts at the distal renal tubule to conserve salt and water at the expense of K+ loss (Ch. 14). Therefore, angiotensin II and aldosterone raise blood pressure by vasoconstriction and by increasing circulating blood volume. Some of the effects of angiotensin at the AT1 receptor do not raise blood pressure; in the endothelium, AT1 receptor stimulation enhances nitric oxide production, resulting in vasodilation and dampening the pressor effects of angiotensin II. Angiotensin II has additional actions at the AT2 receptor that appear to oppose some of those at the AT1 receptor (Fig. 6.2).
Fig. 6.2 Formation and actions of angiotensin II.
Angiotensin II acts on type 1 (AT1) and type 2 (AT2) receptors. Current therapeutic drugs act predominantly to block AT1 receptors. The number of AT2 receptors is low relative to that of AT1 receptors but increases in pathological conditions. ACE, angiotensin-converting enzyme; ADH, antidiuretic hormone; BK, bradykinin, NO, nitric oxide; ROS, reactive oxygen species.
Fig. 6.3 The control of blood pressure via the sympathetic and renin–angiotensin–aldosterone systems.
A fall in cardiac output or blood pressure (yellow box) produces relatively rapid responses mediated by increased sympathetic activity and slower responses mediated by renin–angiotensin–aldosterone mechanisms. The outcomes are increased cardiac stimulation, increased arterial constriction and increased venous return, restoring blood pressure. AT1, angiotensin II type 1 receptor.
The integration of the fast-responding sympathetic nervous system and the slower-responding renin–angiotensin–aldosterone system in response to a fall in blood pressure is shown in Figure 6.3. These mechanisms prevent hypotension due to peripheral pooling of blood on standing and during exercise.
Additional mechanisms involved in controlling vascular tone and blood volume include circulating or local endothelial hormones and metabolites, such as natriuretic peptides, antidiuretic hoemone, prostaglandins, bradykinin, nitric oxide, endothelin and adenosine. Their relative importance may differ in health and disease states.
Aetiology and pathogenesis of systemic hypertension
There is no absolute cut-off between normal and high blood pressure. Blood pressure in all populations is normally distributed with a slight skew because of a small number of individuals with very high blood pressures. The risk of complications is also a continuous variable, increasing as blood pressure rises. Defining a point at which blood pressure is ‘high’ is therefore somewhat arbitrary but it is usually set at values greater than 140/90 mmHg. Using this definition, hypertension is a common condition found in 20–30% of the population of the developed world, with the prevalence increasing with age.
Hypertension does not usually cause symptoms but produces progressive structural changes in the heart and circulation. These include formation of atheroma, microaneurysms on intracerebral blood vessels and remodelling of the muscle in the left ventricle and arterial resistance vessels. These changes predispose to clinical complications that are often referred to as ‘target organ damage’. The principal complications of hypertension are ischaemic heart disease (especially in middle-aged Europeans and Americans), and cerebrovascular disease (especially in Asians and older people) which usually presents as thromboembolic stroke or, less commonly, as cerebral haemorrhage (Ch. 9). Ischaemic complications of hypertension are more likely to occur if it is accompanied by hypercholesterolaemia, diabetes mellitus and smoking. The underlying vascular lesions that occur in hypertension and their resulting complications are shown in Figure 6.4.
Fig. 6.4 Complications of hypertension.
Hypertension causes vascular lesions and damage throughout the body.
Sustained hypertension predisposes to left ventricular muscle hypertrophy (LVH). LVH is an independent risk factor for the complications of hypertension, particularly ischaemic heart disease (since the muscle outgrows its blood supply), heart failure with preserved ejection fraction (Ch. 7) and arrhythmias leading to sudden death (Ch. 8).
If target organ damage or diabetes is present, treatment reduces complications when introduced at blood pressures above 140/90 mmHg. However, if there is no target organ damage treatment may not alter outcome until the systolic pressure is sustained above 160 mmHg, or the diastolic blood pressure is sustained above 100 mmHg.
Hypertension should ideally be confirmed by ambulatory 24 h blood pressure monitoring, unless target organ damage gives a clear indication treatment is necessary. Sometimes, the blood pressure is raised only when the measurement is taken by a doctor or, to a lesser extent, by a nurse. This phenomenon is termed ‘white coat’ or ‘office’ hypertension and appears to carry little risk of complications over the subsequent few years but can eventually lead to sustained hypertension. It can be detected by ambulatory 24 h blood pressure monitoring. White coat hypertension often persists despite drug treatment, which can then result in quite troublesome hypotension away from the surgery or clinic. There is also a phenomenon of ‘masked’ hypertension, when blood pressure is normal in the clinic but high at home. This appears to carry a similar risk of complications as sustained hypertension.
Malignant or accelerated hypertension is an infrequently encountered condition, produced by very high blood pressure or a rapid rise in blood pressure. It is characterised pathologically by arterial fibrinoid necrosis, and identified clinically by the presence of flame-shaped haemorrhages, hard exudates and ‘cotton wool’ spots in the retina or by papilloedema, which can lead to visual disturbance. If untreated, accelerated hypertension usually leads to death from renal failure, heart failure or stroke within 5 years.
Hypertension is usually characterised by increased peripheral arterial resistance, which arises from arteriolar smooth muscle constriction and hypertrophy leaving a smaller vessel lumen and an increase in the wall-to-lumen ratio (vascular remodelling). Cardiac output is often normal in younger people with hypertension, but is usually reduced in the elderly. The cause of the inappropriately raised peripheral resistance is unknown in the majority of people with hypertension, who are said to have ‘essential hypertension’. Essential hypertension probably has a polygenic inheritance, leading to several clinical subtypes with different underlying pathogenic mechanisms. Environmental influences and factors such as diet, level of exercise, obesity and alcohol intake all interact with the genetic programming to determine the final level of blood pressure. There is evidence that reduced renal Na+ excretion plays a central role in the pathogenesis of essential hypertension, and the kidney requires a higher-than-normal blood pressure to maintain a normal extracellular fluid volume. However, the disturbance in essential hypertension is much more widespread than the kidney, with cell membrane abnormalities found in many organs.
Isolated systolic hypertension, usually found in older people, is the consequence of stiffening of large ‘conductance’ arteries. These vessels normally expand to accommodate the blood expelled from the heart in systole, which slows the pulse wave and increases the time taken for it to reach the peripheral resistance vessels. The pulse wave is normally reflected back from the peripheral vessels in diastole, and supports the diastolic blood pressure and therefore coronary artery perfusion. If the compliance of the large arteries is reduced, then the pulse wave reaches the peripheral vessels early and is reflected back in systole. This increases systolic blood pressure and reduces diastolic pressure. In isolated systolic hypertension, coronary artery perfusion can be impaired, while cardiac work is increased.
A secondary underlying cause of high blood pressure, which often has a renal or endocrine origin, can be identified in about 5% of people with hypertension (Table 6.1).
Table 6.1
Principal causes of secondary hypertension
Causes | |
Renal | Renal artery stenosis, glomerulonephritis, interstitial nephritis, arteritis, polycystic disease, chronic pyelonephritis |
Endocrine | Conn’s syndrome (aldosterone excess), Cushing’s syndrome (glucocorticoid excess), phaeochromocytoma (catecholamine excess), acromegaly |
Pregnancy | Pre-eclampsia and eclampsia |
Drugs | Oestrogen, corticosteroids, non-steroidal anti-inflammatory drugs (NSAIDs), ciclosporin |
Not surprisingly, since the cause of hypertension is unclear, treatment cannot be directed precisely at the underlying mechanism(s). Most antihypertensive drugs are vasodilators, and they often modulate the natural hormonal or neuronal mechanisms responsible for blood pressure regulation. Less commonly, a hypotensive action is partially achieved by reducing cardiac output. The principal classes of antihypertensive drugs and their sites of action are shown in Table 6.2 and Figure 6.5.
Antihypertensive drugs
Drugs acting on the sympathetic nervous system
Beta-adrenoceptor antagonists (β-blockers):
Mechanism of action in hypertension: Beta-adrenoceptor antagonists reduce blood pressure in several ways (Fig. 6.6). Selective β1-adrenoceptor antagonists are as effective as non-selective drugs, indicating that β2-adrenoceptor blockade makes little contribution. The more important actions are probably:
Fig. 6.6 Sites of action of the β-adrenoceptor antagonists relevant to their use as antihypertensive agents.
(A) In the heart, the β1-adrenoceptor antagonist drugs reduce stimulation of the β1-adrenoceptors by noradrenaline (NA) and circulating adrenaline (ADR). Presynaptic stimulation of α2-adrenoceptors, which inhibits noradrenaline release, still functions normally. (B) In the kidney, β1-adrenoceptor blockade reduces the activity of the renin–angiotensin system. (C) Some selective β1-adrenoceptor antagonists have hybrid activity: pindolol and celiprolol have intrinsic sympathomimetic activity, acting as partial agonists at β2-adrenoceptors in skeletal muscle blood vessels, leading to vasodilation and reduced peripheral resistance. As partial agonists these drugs reduce heart rate and cardiac output less than full antagonists. Nebivolol may dilate blood vessels more generally by releasing nitric oxide (NO). Carvedilol and labetalol also have α1-adrenoceptor antagonist activity, reducing peripheral resistance.


For further details about β-adrenoceptor antagonists and their many uses, see Chapters 5 and 8, and Box 6.1.
Box 6.1 Clinical uses of β-adrenoceptor antagonists
Treatment of hypertension (this chapter)
Prophylaxis of angina (Ch. 5)
Secondary prevention after myocardial infarction (Ch. 5)
Treatment of heart failure (Ch. 7)
Prevention and treatment of arrhythmias (Ch. 8)
Control of symptoms in thyrotoxicosis (Ch. 41)
Alleviation of symptoms in anxiety (Ch. 20)
Prophylaxis of migraine (Ch. 26)
Topical treatment of glaucoma (Ch. 50)
Alpha-adrenoceptor antagonists (α-blockers):
Examples
α1-adrenoceptor-selective antagonists: doxazosin, prazosin
non-selective antagonists: phenoxybenzamine (irreversible), phentolamine (reversible)
Mechanisms of action: Alpha-adrenoceptor antagonists (often referred to as α-blockers) lower blood pressure by blockade of postsynaptic α1-adrenoceptors, leading to:
When blood pressure falls as a result of using an α-adrenoceptor antagonist, this is detected by arterial baroreceptors. The baroreceptors initiate an increase in sympathetic discharge from the medulla, causing a reflex tachycardia (Figs 6.1 and 6.3). However, because noradrenaline released from cardiac sympathetic nerve terminals also stimulates inhibitory α2-adrenoceptors on the presynaptic sympathetic neuron, the degree of sympathetic stimulation and reflex tachycardia is attenuated (Fig. 6.6A). Selective α1-adrenoceptor antagonists do not block the presynaptic α2-adrenoceptors on sympathetic nerve terminals, and therefore reflex tachycardia is unusual with these compounds.
By contrast, non-selective α-adrenoceptor antagonists block both postsynaptic α1-adrenoceptors and presynaptic α2-adrenoceptors, and their use is accompanied by a marked reflex tachycardia. Non-selective agents now have little place in clinical practice except for the perioperative management of phaeochromocytoma.
Alpha-adrenoceptor antagonists produce a potentially beneficial effect on plasma lipids by increasing high-density lipoprotein (HDL) cholesterol and reducing triglycerides (Ch. 48). Whether this has any relevance for the prevention of atheroma in individuals with hypertension is uncertain. Selective α1-adrenoceptor antagonists are also used to treat the symptoms of bladder outlet obstruction (Ch. 15).
Pharmacokinetics: Selective α1-adrenoceptor antagonists are well absorbed from the gut and undergo extensive first-pass metabolism and subsequent elimination by the liver. The compounds differ principally in their half-lives and, therefore, duration of action; for example, prazosin has a half-life of 3 h whereas doxazosin has a half-life of 9–12 h.
Centrally acting antihypertensive drugs
Selective imidazoline receptor agonists:
Mechanism of action: Imidazoline I1 receptors are important for the regulation of sympathetic drive (Figs 6.1 and 6.7). They are concentrated in the rostral ventrolateral medulla, a part of the brainstem involved in control of blood pressure. Increased neuronal activity in this area, either through baroreceptor stimulation or by direct stimulation of I1 receptors by moxonidine, will decrease sympathetic outflow, which results in a fall in blood pressure with no reflex tachycardia. Unlike other centrally acting drugs (clonidine and methyldopa), moxonidine has a low affinity for presynaptic α2-adrenoceptors.
Fig. 6.7 Mechanisms of centrally acting antihypertensive drugs.
These drugs act on the same medullary centres that respond to raised blood pressure (Fig. 6.1). Methylnoradrenaline and clonidine stimulate α2-adrenoceptors in the nucleus of the tractus solitarius (NTS). Moxonidine, and possibly also clonidine, act on imidazoline I1 receptors in the rostral ventrolateral medulla (RVLM). VN, vagal nucleus (cardioinhibitory centre).
Pharmacokinetics: Moxonidine is well absorbed from the gut, and its principal route of elimination is the kidney. It has a short half-life (2–3 h) but a prolonged duration of action, which may reflect its high affinity for I1 receptors.
Centrally acting α2-adrenoceptor agonists:
Unwanted effects limit the use of the centrally acting α2-adrenoceptor agonists, although methyldopa is a drug of choice in the treatment of hypertension in pregnancy (see below).
Mechanisms of action: The α2-adrenoceptor agonists act at presynaptic autoreceptors in the central nervous system (CNS) to reduce sympathetic nervous outflow and increase vagal outflow from the medulla (Fig. 6.7). This reduces both peripheral arterial and venous tone.
Methyldopa is a prodrug that is metabolised in the nerve terminal as a ‘false substrate’ in the biosynthetic pathway for noradrenaline, to produce α-methylnoradrenaline, a potent α2-adrenoceptor agonist. Clonidine is a direct-acting α2-adrenoceptor agonist that is also an agonist at imidazoline I1 receptors (see above). Clonidine has some peripheral postsynaptic α1-adrenoceptor agonist activity, which produces direct peripheral vasoconstriction; this initially offsets some of the central blood pressure-lowering effect.
Pharmacokinetics: Methyldopa is incompletely absorbed from the gut and undergoes dose-dependent first-pass metabolism, with an elimination half-life of 1–2 h. Clonidine is completely absorbed from the gut and has a half-life of about 24 h.



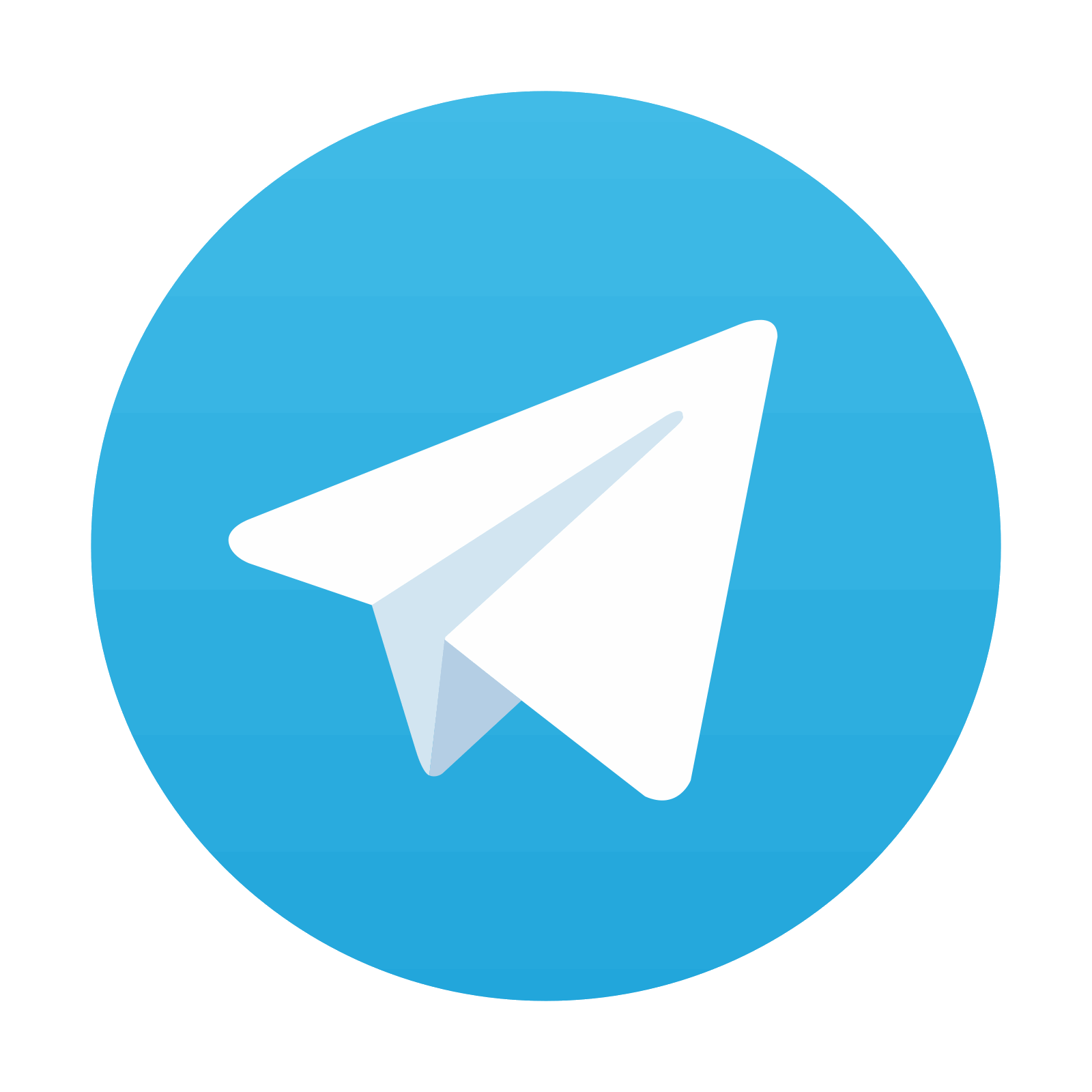
Stay updated, free articles. Join our Telegram channel
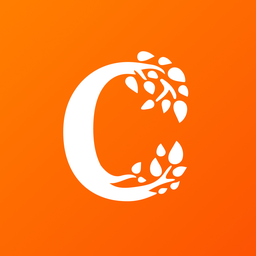
Full access? Get Clinical Tree
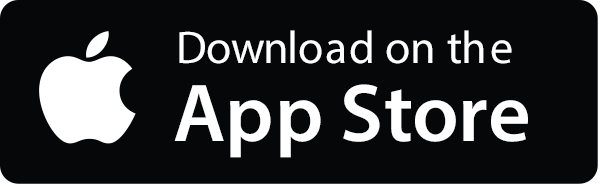
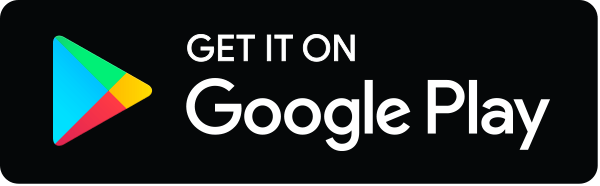