
TABLE 29.1 Machine classification, design, and application | |||||||||||||||||||||||||
---|---|---|---|---|---|---|---|---|---|---|---|---|---|---|---|---|---|---|---|---|---|---|---|---|---|
|
is generated using direct current, radiofrequency or microwave energy. Once the electrons are accelerated to the desired energy, the beam can then be managed by magnetic fields to achieve such results as increasing its size, changing its shape, or scanning over a predetermined area.
TABLE 29.2 Units and conversion factors in radiation | ||||||||||||
---|---|---|---|---|---|---|---|---|---|---|---|---|
|

(0.4-8 kGy), the changes are usually limited to nutritional quality, odor, taste, and color; however, the degree of change(s) is mostly related to the overall dose delivered (either no change up to significant change). The interaction with biological liquids, tissues, or drug components can also produce changes. At the doses used in the sterilization process, changes may occur that cause a degradation of the drug content or functional aspects of the biological liquids or tissue such as protein-based substances (eg, collagen, human or recombinant albumin, tissue). Radiation may modify these by breaking of the protein chains and disrupting the ability of the amino acid to combine with other polymers, which can change the function of the material. Fluids may undergo changes when exposed to radiation, the type and degree of which are based on the chemical makeup of the fluid, the dose, and the dose rate of the radiation process. As with all other materials, free radicals are produced that combine to result in a change in the fluid. The most observed and easiest to quantitate change is that of pH. Reactions in fluids are completed in less time than those in solid materials, thus resulting in less additional change over time.

that exhibit a shoulder effect before exponential inactivation, which may be due to repair mechanisms. Examples of these organisms are Deinococcus (originally Micrococcus) radiodurans10 and radiation-resistant mutants of Salmonella ser Typhimurium.4 These curves are indicative of typical homogeneous populations.


may increase organism resistance due to repeated contact with chemical disinfectants. This increased resistance may require a radiation pretreatment of raw materials or components to mitigate resistance developed by the microbial flora. Alternatively, another sterilization method might be selected for pretreatment of raw materials or components, depending on the microorganism observed and the root cause of the increased radiation resistance.
TABLE 29.3 Factors that modify radiation resistance | |||||||||||||||||||||||||||||||||||||||||||||||||||||||||||||||||||||||||||||||||||||||
---|---|---|---|---|---|---|---|---|---|---|---|---|---|---|---|---|---|---|---|---|---|---|---|---|---|---|---|---|---|---|---|---|---|---|---|---|---|---|---|---|---|---|---|---|---|---|---|---|---|---|---|---|---|---|---|---|---|---|---|---|---|---|---|---|---|---|---|---|---|---|---|---|---|---|---|---|---|---|---|---|---|---|---|---|---|---|---|
|

bioburden population count or bioburden resistance. Method 1 and VDmax are both based on the bioburden count that fall within the microorganism standard distribution of resistance (SDR). The SDR specifies resistances of microorganisms in terms of D10 values and the probability of occurrence of values in the total population.29 For families of products where the product microbial resistance is less than or equal to the SDR, method 1 may be used to validate a lower sterilization dose; however, if groups of products need to run together at a standardized dose or
products are of high value (eg, implants), then the VDmax method that requires less samples may be more favorable. If the product microbial resistance is not less than or equal to the SDR, then the method must be based on the determination of microbial resistance, which would require the use of method 2. The method of choice may also be governed by the functional dose limitations of the product due to material compatibility that would require a dose establishment method capable of qualifying a lower sterilization dose such as method 1 or method 2.
TABLE 29.4 Radiation resistance of microorganisms and other biological units | ||||||||||||||||||||||||||||||||||||||||||||||||||||||||||||||||||||||||||||||||||||||||||||||||||||||||||||||||||||||||||||||||||||||||||||||||||||||||||||||||||||||||||||||||||||||||||||||||||||||||||||||||||||||||||||||||||||||||||||||||||||||||||||
---|---|---|---|---|---|---|---|---|---|---|---|---|---|---|---|---|---|---|---|---|---|---|---|---|---|---|---|---|---|---|---|---|---|---|---|---|---|---|---|---|---|---|---|---|---|---|---|---|---|---|---|---|---|---|---|---|---|---|---|---|---|---|---|---|---|---|---|---|---|---|---|---|---|---|---|---|---|---|---|---|---|---|---|---|---|---|---|---|---|---|---|---|---|---|---|---|---|---|---|---|---|---|---|---|---|---|---|---|---|---|---|---|---|---|---|---|---|---|---|---|---|---|---|---|---|---|---|---|---|---|---|---|---|---|---|---|---|---|---|---|---|---|---|---|---|---|---|---|---|---|---|---|---|---|---|---|---|---|---|---|---|---|---|---|---|---|---|---|---|---|---|---|---|---|---|---|---|---|---|---|---|---|---|---|---|---|---|---|---|---|---|---|---|---|---|---|---|---|---|---|---|---|---|---|---|---|---|---|---|---|---|---|---|---|---|---|---|---|---|---|---|---|---|---|---|---|---|---|---|---|---|---|---|---|---|---|---|---|---|---|---|---|---|---|---|---|---|---|---|---|---|---|
|
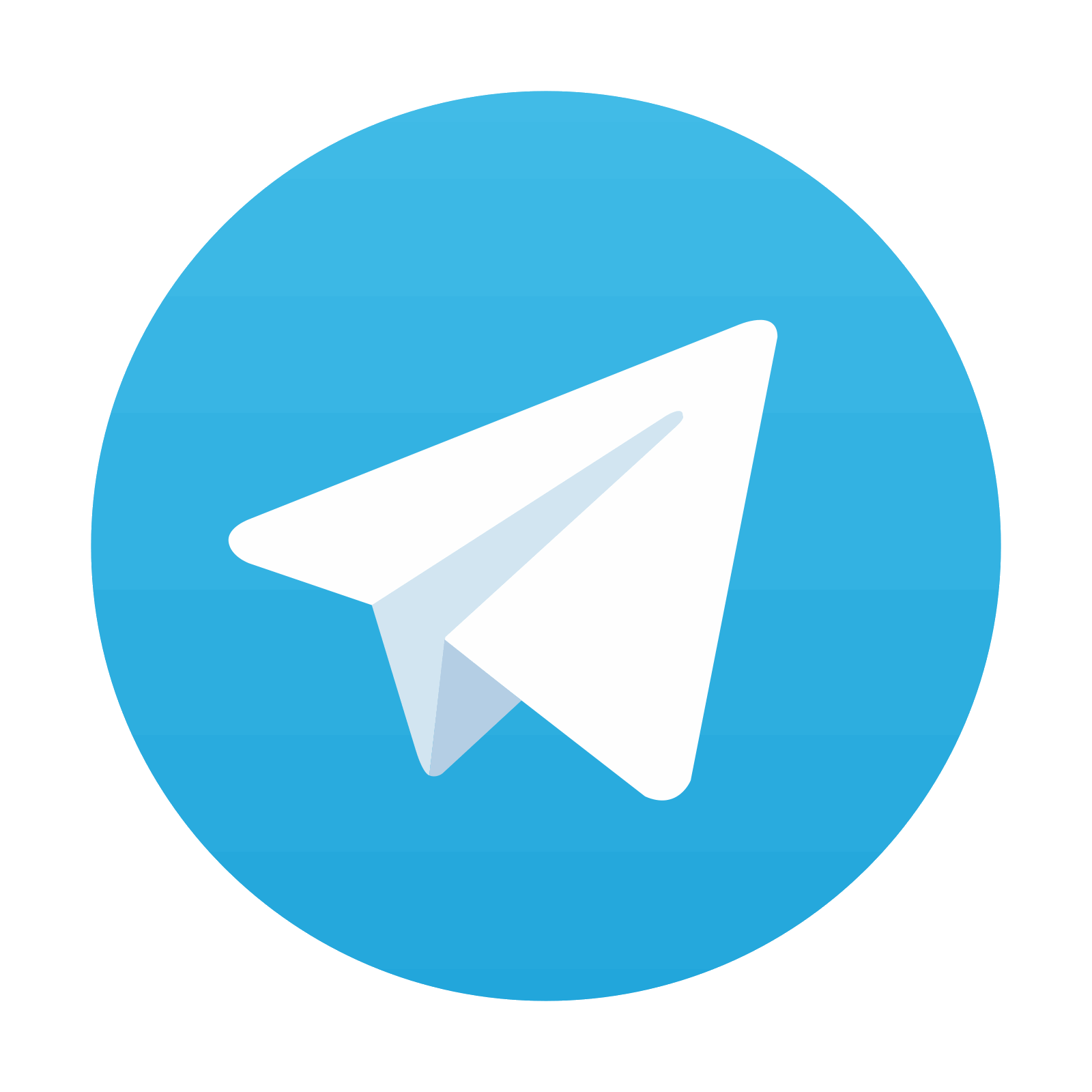
Stay updated, free articles. Join our Telegram channel
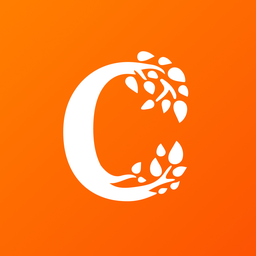
Full access? Get Clinical Tree
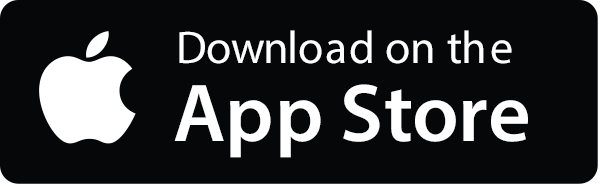
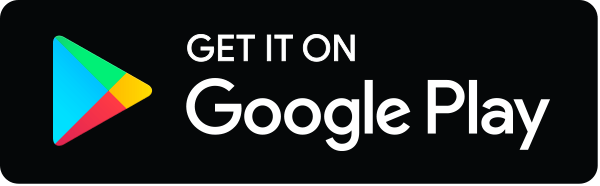