Fig. 17.1
Reactogenicity of adjuvant-treated skin. The low back skin of BALB/c mice was treated with a laser defined in 17.3.2.1, TLR agonist-based adjuvants (MPL, R837, CpG), depot adjuvants (alum, Montanide ISA 720), and combinatorial adjuvants (LVA/MPL, LVA/CpG, MPL/CpG, MPL/R837, MPL/alum). Five days later, photos of adjuvant-treated sites were taken (upper panel; scale bar, 2 mm), followed by histological examination (lower panel; scale bar, 100 μm). The lesion in the skin is outlined by a circle in the upper panel. Arrows in the lower panel in Montanide ISA 720-injected skin point to void bulbs preoccupied by the water-in-oil adjuvant
17.3.2 Laser Vaccine Adjuvants
Apart from conventional adjuvants, we, alongside others, took a totally different approach in developing physical type laser adjuvants to specifically boost skin vaccination (Kashiwagi et al. 2013, 2014; Wang et al. 2014; Chen et al. 2010). Two kinds of laser adjuvants have been developed to boost ID vaccination with the first causing no skin damage or inflammation and the second generating microscopic skin damage (Kashiwagi et al. 2013; Wang et al. 2014; Chen et al. 2010). The unique feature of the laser adjuvants is the superb local safety and no potential systemic or long-term side effects since no foreign materials are injected into the body with laser adjuvantation (Chen and Wu 2011; Chen et al. 2013; Kashiwagi et al. 2013, 2014; Wang et al. 2014; Chen et al. 2010).
17.3.2.1 Laser Vaccine Adjuvant (LVA)
A Q-switched 532 nm Nd: YAG laser was first demonstrated to possess adjuvant effects (Chen et al. 2010). Laser illumination (0.3 W, 2 min) of a small area of the skin (<1 cm2) followed by ID injection of model antigen ovalbumin (OVA) into laser-illuminated site significantly enhanced OVA-specific humoral immunity without skin damage or inflammation (Chen et al. 2010). LVA is also able to augment immune responses induced by seasonal influenza vaccine, recombinant malarial liver-stage antigen 1, hepatitis B surface antigen (HBsAg), and nicotine vaccine (Chen and Wu 2011; Chen et al. 2010). Since laser at 532 nm can be strongly absorbed by melanin, laser adjuvant effect might vary with skin colors. To overcome this, near-infrared (NIR) laser was also tested for its adjuvant effect (Kashiwagi et al. 2013). Similar to 532 nm laser, NIR laser significantly enhanced OVA and influenza vaccine-induced immune responses (Kashiwagi et al. 2013). Laser adjuvants can be used alone or combined with other conventional adjuvants to synergistically or additively augment vaccine-induced immune responses (Chen and Wu 2011; Chen et al. 2012). We showed that a combination of LVA and MPL augmented NicAb titer by 33-fold, while LVA or MPL only increased NicAb titers by 4-fold or 13-fold, respectively (Chen et al. 2012). Importantly, the combination did not induce overt skin reactions (Fig. 17.1), holding great promise for clinical use (Chen and Wu 2011; Chen et al. 2012).
The mechanism underlying laser-induced adjuvanicity is not completely understood, but it is clear that tissue damage or inflammation is not responsible for the adjuvant effect, in contrast to the majority of conventional adjuvants (Chen and Wu 2011; Kashiwagi et al. 2013, 2014; Chen et al. 2010). It is reported that laser treatment at 2–20-fold higher power can induce production of extracellular heat-shock protein (HSP) 70 and inflammatory response (Mackanos and Contag 2011). But, neither HSP70 nor proinflammatory cytokines were detected at the site of laser illumination (Chen and Wu 2011; Chen et al. 2010). Rather, we found that laser treatment accelerated the motility of APC, presumably due to alteration of the dense connective tissue of the skin (Chen and Wu 2011; Chen et al. 2010). The loosely connected micro-architecture of the skin after laser illumination permits free movement of APCs in the dermal tissue and allows them to survey a greater area and better sample antigens (Chen and Wu 2011; Chen et al. 2010). The impact on APCs was also found with the NIR laser, although the enhanced recruitment and migration of APCs may be ascribed to local cytokine and chemokine production (Kashiwagi et al. 2013, 2014). Further investigation of the underlying mechanism will help to improve and apply the laser adjuvant in the clinics.
17.3.2.2 Non-ablative Fractional Laser (NAFL)
NAFL represents a second type of laser adjuvant (Wang et al. 2014). Sterile inflammation is well known to be induced by “danger” signals released from dying cells as a natural response to pathogen invasion or tissue damage (Chen and Nunez 2010). This inflammatory response also plays a crucial role in the adjuvant effect of alum and MF59 because the adjuvants induce cell death at the site of inoculation (Vono et al. 2013; Lambrecht et al. 2009; Kool et al. 2008). But the sterile inflammation provoked by the adjuvants often causes significant local reactions due to too much unwanted cell death that stimulates bulk, persistent skin inflammation as indicated in Fig. 17.1. Utilization of NAFL is not to detour sterile inflammation, but to reduce the side effects of this process by segregation of the sterile inflammation into hundreds of micro-zones (Wang et al. 2014). Each of these micro-inflammation zones can be quickly resolved before it can spread into the nearby tissue, provided that distances between nearby zones are large enough (Wang et al. 2014). Our investigations clearly demonstrated that quick resolution of the inflammation in each micro-zone effectively averted skin lesion while evoking transient, yet sufficient innate immunity to augment immune responses against the vaccine (Wang et al. 2014). Moreover, the laser treatment does not damage the SC layer, so that the barrier function of skin was well preserved, minimizing the risk of skin infections (Wang et al. 2014). The superb safety of NAFL is further warranted as the technology has been broadly employed in the clinics or at home for cosmetic wrinkle removal and skin resurfacing in the past decade (Preissig et al. 2012). The 1,410 nm laser works in a stamp/scanning fashion and is equally effective regardless of skin colors (Wang et al. 2014). It emits high-energy laser beams onto skin surface and generates an array of self-healing microthermal zones (MTZ) beneath skin surface (Wang et al. 2014). The dying cells within MTZs release “danger” signals also called damage associated molecule patterns (DAMPs) to alert the immune system (Wang et al. 2014). Thus, each MTZ can be regarded as a mini adjuvant zone and hundreds of such mini adjuvant zones could be very effective in augmenting vaccine-induced immune responses (Wang et al. 2014). Indeed, illumination of the inoculation site with NAFL prior to ID vaccination significantly enhanced immune responses against OVA, HBsAg, and seasonal influenza vaccine (Wang et al. 2014). The adjuvant effect has been also confirmed in swine, in which the immune responses are augmented by eight-, five-, and twofolds for HBsAg, influenza vaccine, and nicotine vaccine, respectively (Wang et al. 2014).
Intravital confocal microscopy revealed an increase in the motility of APCs around each MTZ soon after laser illumination (Wang et al. 2014). These APCs were actively recruited into the vicinity of MTZs, forming a micro-sterile inflammation array (Wang et al. 2014). The recruitment is likely directed by various chemokines including CCL2, CCL3, CCL7, CCL20, CXCL9, CXCL10, and CXCL2 that are expressed at high levels at the inoculation site following NAFL treatment (Wang et al. 2014). Over a 48 h period, these APCs were activated around individual MTZs, and upon activation, the cells migrated into the draining lymph nodes (Wang et al. 2014). Accelerated emigration of activated APCs into the draining lymph nodes was crucial for augmentation of vaccine-induced immunity as well as for reduction of local inflammation (Wang et al. 2014). In this regard, a smaller number of activated APCs at the inoculation site can speed up resolution of inflammation, whereas a higher number of mature APCs in draining lymph nodes are pivotal for heightened immune responses. One of the crucial “danger” signals was recently identified to be double-strand DNA (dsDNA) released from laser-damaged cells (Wang et al. 2014). Thus, mice deficient in STING, a key protein in DNA sensing pathway, could not respond to NAFL treatment and failed to augment immune responses against influenza vaccines (Wang et al. 2014). In comparison, mice deficient in other immune pathways, including TLRs and inflammasome, responded to NAFL treatment similarly to wild-type mice (Wang et al. 2014).
17.4 Adjuvant-Incorporated ID Nicotine Vaccination
Several adjuvants, like LVA and MPL adjuvants that cause little skin irritation and a highly potent MPL/CpG adjuvant, were tested in ID nicotine vaccination. As can be seen in Fig. 17.2a, incorporation of LVA only in the primary immunization in a 3-dose regimen significantly increased NicAb titer by 2.5 and 18.5 times as compared to the same doses of ID and IM immunization, respectively. Two ID immunizations in the presence of MPL induced 5.0 times higher NicAb titer than 3 ID immunizations or 6.8 times higher NicAb titer than 7 IM immunizations (Fig. 17.2a). Two ID immunizations in the presence of MPL/CpG further increased NicAb titer that was 9.3 times higher than 3 ID immunizations and 12.6 times higher than 7 IM immunizations (Fig. 17.2a). NicAb titer after 3 ID immunizations in the presence of LVA or 2 immunizations in the presence of MPL or MPL/CpG was 1.9, 3.5, or 6.9 times higher than that induced by 4 IM immunizations in the presence of alum adjuvant, respectively (Fig. 17.2a). The latter is a vaccination regimen similar to the clinical trial. Incorporation of adjuvants into ID immunization also significantly prolonged a high NicAb titer for more than 3 months as compared to ID group (Fig. 17.2a). Increased NicAb titers after incorporation of adjuvants into ID immunization more significantly inhibited nicotine entry into the brain after nicotine challenge (Fig. 17.2b). Decreases in brain nicotine levels by 34 %, 48 %, or 62 % were attained after incorporation of LVA, MPL, and MPL/CpG into ID immunization, respectively (Fig. 17.2b). Among the 3 adjuvants tested, MPL/CpG adjuvant is the most potent and brain nicotine levels after 2 ID immunizations in the presence of MPL/CpG were only half of those after 4 IM immunizations in the presence of alum adjuvant (Fig. 17.2b). As compared to ID vaccination alone, serum nicotine level was increased by 167 %, 130 %, and 294 % after incorporation of LVA, MPL, and MPL/CpG adjuvant, respectively (Fig. 17.2c). Serum nicotine level was comparable between LVA + ID and alum + IM group (Fig. 17.2c). The more significant inhibition of nicotine entry into the brain with a fewer doses of MPL/CpG-incorporated ID immunization strongly indicates the advantages of MPL/CpG adjuvant in adjuvantation of nicotine vaccines.
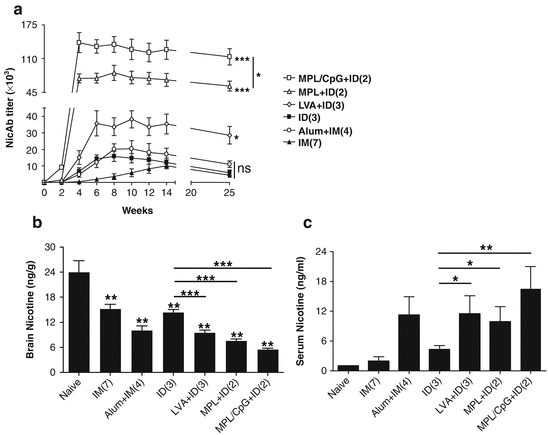
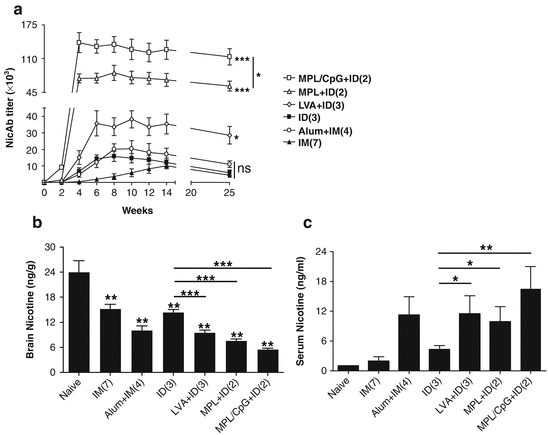
Fig. 17.2
Superior ID nicotine vaccine immunization with various adjuvants. (a) BALB/c mice were IM immunized with Nic-KLH alone (filled triangle) or in combination with alum (circle) or ID immunized with Nic-KLH alone (filled rectangle) or in combination with LVA (diamond), MPL (triangle), and MPL/CpG (rectangle). The number of immunizations is shown in the parentheses behind the group name. Serum NicAb titer was measured one day before each immunization and 2 and 13 weeks after the last immunization. NicAb titer in the alum + IM group was compared with the IM group, while NicAb titers in LVA + ID, MPL + ID, and MPL/CpG + ID group were compared with the ID group. (b, c) Brain and blood nicotine levels following nicotine challenge. Nonimmunized or nicotine vaccine-immunized mice were intravenously challenged with 0.01 mg/kg nicotine, and 5 min later, brain and blood were harvested and brain (b) and serum nicotine levels (c) were quantified by gas chromatography. Two-tailed student t-test was used to compare difference between various immunization groups and nonimmunized control group or between LVA-, MPL-, and MPL/CpG-incorporated ID immunization group and ID immunization alone in (b). One-tailed Mann-Whitney test was used to compare difference between LVA-, MPL-, and MPL/CpG-incorporated ID immunization and ID immunization alone in (c). n = 4–6. *, **, ***, p < 0.05, 0.01, and 0.001, respectively
17.5 Novel Skin Delivery Technologies
Apart from safe adjuvants for skin vaccination, how to deliver vaccines and adjuvants into the skin without overt skin reactions remains a significant challenge. This is particularly true for nicotine vaccines considering nicotine vaccines require potent adjuvants to be effective. For instance, the aforementioned MPL/CpG adjuvant profoundly improved immunogenicity of ID nicotine vaccine, yet caused severe local reactions (Fig. 17.1). Aside from adjuvant-induced local reactions, it is well documented in the clinics that ID vaccination alone often causes more frequent and severer local reactions than IM vaccination (Leroux-Roels et al. 2008; Sticchi et al. 2010). For instance, the live-attenuated Mycobacterium bovis Bacillus Calmette-Guerin (BCG) vaccine induced severe local reactions that ended with permanent scars in more than 90 % vaccinees (Jeena et al. 2001). ID rabies vaccine induced much higher rates of erythema, pruritus, and other local reactions than IM vaccination (Lang et al. 1999). ID influenza vaccine induced an overall 30–75 % erythema, induration, edema, and pruritus at local injection site, while IM injection induced only <10 % of these local reactions (Leroux-Roels et al. 2008). Because of more unwanted skin irritation associated with ID vaccination, BCG vaccine, rabies vaccine, and seasonal influenza vaccine are the only vaccines approved for ID delivery, among which BCG vaccine has to be administered into the dermal tissue to avoid abscesses and the other two vaccines are delivered into the dermal tissue for dose sparing and cost saving (Leroux-Roels et al. 2008; Jeena et al. 2001; Lang et al. 1999). Thus, development of novel ID or skin delivery technologies with minimized local reactions are urgently needed. Next we introduce recent advances in developing reliable ID delivery technologies and novel skin delivery technologies that are needle-free, painless, and potentially lesion-free.
17.5.1 ID Immunization
Due to the thin skin tissue, special techniques are required to accurately deliver vaccines into the skin. Currently, Mantoux technique, developed by Charles Mantoux in the early twentieth century, has been used for ID delivery of BCG vaccine and tuberculin for tuberculosis (TB) prevention and diagnosis, respectively (Roth et al. 2005). For accurate ID delivery, needle bevel in Mantoux method is inserted at a 5–15° angle into the skin, and after advancing ~3 mm until the entire bevel is covered by the skin, vaccine or tuberculin is slowly injected and a tense, pale wheal will appear around the needle bevel (Kim et al. 2012a). Mantoux technique has been also used for ID delivery of rabies vaccine. Although Mantoux technique can accurately deliver vaccines into the skin, this method requires specially trained personnel and faces significant challenges for use in resource-poor areas (Kim et al. 2012a). Mantoux technique also generates pain because relatively big needles (26th–27th gauge) are used to avoid the curving of the needle during insertion and to ensure the right positioning of the needle bevel within the dermal tissue. Mantoux-based ID injection hasn’t been broadly practiced for mass immunization in the clinics despite the advantage of improved vaccine immunogenicity so far.
To facilitate reliable ID injection, an ID adapter is designed to fit traditional 1 cc syringe and ½ inch needle (Norman et al. 2014). By sitting around the ½ inch needle, the ID adapter guides the injection depth and angles in avoid of needle penetration across the dermal layer. The ID adapter was approved by FDA in 2013 for readily ID injection with minimal training. A microneedle (MN)-based device, called MicronJet 600 (NanoPass), equipped with three hollow pyramid-shaped MNs, each with 250 μm tall, is also developed to replace hypodermic needles to fit with traditional syringes for readily ID injection (Donnelly et al. 2010).
In 2009, a microinjection technique, called ID microinjection system (SoluviaTM, BD), was approved for delivering a reduced dose of seasonal influenza vaccine in adults (Leroux-Roels et al. 2008; Laurent et al. 2007). The microinjection system is a prefilled syringe equipped with an ultrashort (1.5 mm) and ultrafine (30th gauge) needle (Laurent et al. 2007). Different from Mantoux technique, the needle in the microinjection system is perpendicularly inserted into the skin. The human skin is about 2–3 mm in thickness and the microinjection system can accurately deliver vaccine into the dermal tissue with minimal training. In addition, an ~40 % thinner needle in the microinjection system causes less pain during insertion as compared to traditional needles. More than 80 % adults reported no or hardly any pain at the time of microinjection, as compared to ~75 % reported obvious pain in Mantoux-based ID injection (Leroux-Roels et al. 2008; Laurent et al. 2007; Kenney et al. 2004; Belshe et al. 2004). It also causes less pain when compared with IM injection (Leroux-Roels et al. 2008; Laurent et al. 2007; Kenney et al. 2004; Belshe et al. 2004). The microinjection system has been approved for a reduced dose of seasonal influenza vaccine in Europe and the United States today. The clinical test of using the microinjection system in place of a traditional needle to deliver tuberculin has been completed (trial #NCT01611844). Due to the minimal training and pain, the microinjection system holds great promise for ID delivery of vaccines, like hepatitis B vaccine, inactivated polio vaccine, yellow fever vaccine, hepatitis A vaccine, and the like.
Besides the microinjection system, an ID Needle-free Injection System (PharmaJet) has been developed as an alternative to Mantoux-based ID injection (Kwilas et al. 2014). The PharmaJet ID System uses high pressure to generate a narrow, high-velocity fluid to penetrate and deposit liquid vaccines into the dermal tissue (Kim et al. 2012a; Kwilas et al. 2014). To eliminate contamination risks, the contact part with patients in the PharmaJet ID system is a sterile, single-use, disposable needle-free syringe. This system represents the first FDA-approved needle-free injection system that greatly reduces biohazardous sharp waste in association with vaccination. Besides ID injection, PharmaJet needle-free injection system has been also approved as an alternative to traditional syringes and needles for subcutaneous or IM injection.
17.5.2 Novel Technologies for Lesion-Free Skin Vaccination
The aforementioned ID delivery technologies largely ease the technical hurdles of ID injection. Yet, these technologies deliver liquid vaccines into the dermal tissue, causing skin wheals, and are not absolutely painless due to the existence of nerve ends in the dermis. Although only 0.1 ml vaccine liquids are delivered into the dermal tissue (vs. 0.5 ml in IM vaccination), significant skin morphology change occurs to accommodate the vaccine liquids (Laurent et al. 2007). Moreover, these technologies don’t necessarily limit local reactogenicity of vaccines and adjuvants. We next introduce a micro-fractional epidermal powder delivery (EPD) for needle-free, painless vaccination, concurrent with little local reactogenicity (Chen et al. 2014).
It is well known that skin injury is healed by expansion of surrounding healthy epithelial cells into injured area (Shaw and Martin 2009). The larger the skin injury is, the longer the healing takes. On the contrary, if separated micro-injuries are generated in the skin surface with an equivalent area to a bulk injury, these micro-injuries would be healed much quicker than the bulk injury (e.g., days vs. weeks). This fully repairing capacity forms the basis of ablative fractional laser- and MN-based cosmetic skin resurfacing (Manstein et al. 2004). We extend the quick recovery of micro-injuries to transdermal vaccine delivery aimed at minimizing vaccine-induced local reactions. One of these technologies is called micro-fractional epidermal powder delivery, abbreviated as EPD, based on pretreatment of the skin with ablative fractional laser or MN to generate an array of microchannels (MC) in the epidermis followed by topical application of powder vaccine-coated array patches to deliver vaccines into skin via MCs (Chen et al. 2014). Our investigation shows that EPD generates more than 80 % delivery efficiency within 1 h (Chen et al. 2014). Efflux of interstitial fluid into the MCs probably plays a crucial role in laser- or MN-based EPD (Chen et al. 2014). It can be envisioned that topically applied vaccine powder would be dissolved by the efflux fluid, and after dissolution, the vaccine diffuses from patches into MCs and then the surrounding tissue along the concentration gradient (Chen et al. 2014). Laser- and MN-generated MCs can be quickly healed by the surrounding normal tissue, thus warranting lesion-free (Chen et al. 2014). While profoundly reducing skin reactions, fractional delivery did not compromise vaccine immunogenicity and adjuvant potency (Chen et al. 2014). As mentioned early, combined MPL and CpG vigorously augmented ID nicotine vaccination, but it caused unacceptable skin lesion (Chen et al. 2012). If the adjuvant is delivered via the EPD technology, the skin lesion is expected to be largely averted. As can be seen in Fig. 17.3, ID delivery of lipopolysaccharide (LPS), similar in structure but more potent than MPL, and CpG adjuvants (LPS/CpG) induced significant local reactions, like erythema, swelling, and ulceration, which peaked on day 4 in mice (Fig. 17.3a) or day 2 in pigs (Fig. 17.3b) (Chen et al. 2014). Although the skin was gradually healed, it was unable to fully recover during the experimental period, as evidenced by the obvious skin morphology change observed 21 days later in mice and 35 days later in pigs (Fig. 17.3). In contrast, EPD of LPS/CpG induced minimal local reactions with little erythema, swelling, or ulceration, and the skin completely recovered within 2–3 weeks (Fig. 17.3). Similarly, ID delivery of the highly reactive BCG vaccine induced severe local reactions, while EPD of BCG vaccine induced minimal local reactions and the skin obtained a full recovery within 10 days (Chen et al. 2014). In addition to the micro-fractional delivery, reduced local reactions and a full skin recovery following EPD may also be ascribed to a slow release of vaccines and adjuvants from laser-generated MCs (Chen et al. 2014). In accordance to this, we found a significant amount of AF647-OVA remained inside MCs and slowly released for >7 days (Chen et al. 2014). In contrast, ID injection acutely releases all vaccine/adjuvant content into the skin and induces robust inflammation at the inoculation site. The local reactions following ID injection occur in the entire skin surface with a few millimeters in diameter, which takes much longer time and is more difficult for new tissue to grow and replace the damaged skin (Chen et al. 2014).
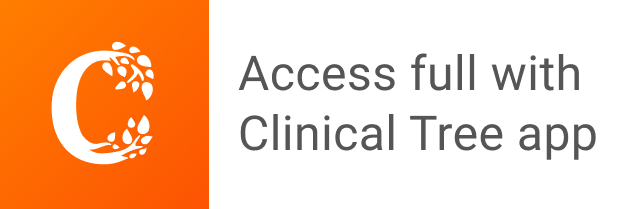