Fig. 5.1
Proposed mechanisms of oxidative stress by redox-inactive toxic metals
5.2 Lead
Lead is a bluish-gray metal found naturally in the earth’s crust. Because of its versatility, lead has been widely used since civilization in paints and dyes, glazed ceramics, gasoline as an antiknock additive, leaded battery, and lead alloys and even to sweeten wine (ATSDR 2007). Humans are exposed to lead through inhalation of lead bound to dust and chemicals and ingestion of lead-contaminated food and drinking beverages. Once it enters the body, lead circulates in the blood and reaches various target organs. In adults, more than 90 % of the total body burden of lead is stored in the skeleton (Saltzman et al. 1990). Although lead in the bones may not be readily toxic, it can be released out of the bones and redistributed to the blood during periods of increased bone resorption such as pregnancy or aging (Hu et al. 1998, 2007). A recent National Toxicology Program (NTP) review concluded that there is sufficient evidence that even low-level exposures for a long period are associated with adverse health effects including neurological, cardiovascular, and renal outcomes (NTP 2012).
5.2.1 Lead and Oxidative Stress
Because lead is a redox-inactive metal, it is known to induce oxidative stress through indirect mechanisms including depletion of thiol groups and damage to antioxidant defense systems. Glutathione (GSH) is a tripeptide containing the thiol group on the cysteine residue and plays an important role in the protection of cells against oxidative damage (Dickinson and Forman 2002). Lead shows a high affinity for GSH, which leads to a reduction in GSH levels and activity in the liver, brain, lungs, and eye lens (Neal et al. 1999; Samarghandian et al. 2013; Sandhir et al. 1994; Sandhir and Gill 1995). Glutathione peroxidase (GPx), catalase (CAT), and superoxide dismutase (SOD), which enzymatically scavenge free radicals, are also potential targets for lead (Farmand et al. 2005; Ni et al. 2004). Lipid peroxidation has also been reported as an effect of lead-induced oxidative stress (Lawton and Donaldson 1991; Yiin and Lin 1995). Numerous epidemiologic studies have also shown associations between lead exposure and markers of oxidative stress such as malondialdehyde (MDA) and γ-glutamyltransferase (GGT), even at low-level exposure in the general population. MDA is used as a biomarker of lipid peroxidation as ROS induce MDA by degrading polyunsaturated fatty acids (Del Rio et al. 2005). Dose-dependent associations between lead exposure and MDA were found in lead-exposed workers, urban adolescents, or children living near a landfill (Ahamed et al. 2006; Cabral et al. 2012; Kasperczyk et al. 2013; Moro et al. 2010; Oktem et al. 2004). Serum GGT has been proposed to be an oxidative stress marker given its role in metabolizing extracellular GSH and providing cysteine for intracellular GSH synthesis (Lee et al. 2004). Lee et al. examined adult participants in the third US National Health and Nutrition Examination Survey (NHANES III) and found strong dose-dependent associations of blood lead with serum GGT as well as other serum biomarkers of antioxidant vitamins (carotenoids, vitamin C, and vitamin E) (Lee et al. 2006).
Lead is also known to increase oxidative stress by interacting with heme biosynthesis. Over 99 % of the lead in blood is partitioned predominantly within red blood cells (RBCs), where most lead is bound to δ-aminolevulinic acid synthetase (δ-ALAS) (Bergdahl et al. 1997). Lead can inhibit δ-ALAS activity due to its high affinity for thiol groups, which results in accumulation of δ-ALA. In lead poisoning, ALA undergoes auto-oxidation and generates superoxide radical (O2 •−), hydrogen peroxide (H2O2), and hydroxyl radical (•OH) (Bechara 1996), which was also evidenced by the inhibition of antioxidant enzymes (e.g., SOD and CAT) (Monteiro et al. 1986).
5.2.2 Lead and Ototoxicity
Hearing loss is a degenerative disease which results from cell death in the cochlea, including degeneration of the organ of Corti, ganglion cell loss, strial atrophy, and basilar membrane stiffness (Liu and Yan 2007). Oxidative stress in mitochondria and reduced blood flow in the cochlea play a pivotal role in the degeneration of the cochlea (Le Prell et al. 2007; Seidman et al. 2004), and lead-induced oxidative stress potentially through mechanisms discussed above may lead to ototoxicity. Other potential mechanisms include neuro-ototoxic effects of lead on the auditory brainstem and cochlea (Bertoni and Sprenkel 1988; Lasky et al. 1995; Yamamura et al. 1989), inhibition of ion flow through calcium channels (Audesirk 1993; Garza et al. 2006), and disruption of plasma membrane calcium pumps, resulting in loss of sensory hair cells and hearing loss (Brini 2009; Schultz et al. 2005; Shull et al. 2003; Spiden et al. 2008).
Tuncel et al. examined ototoxic effects of lead acetate (inorganic lead) and tetraethyl lead (organic lead) using direct ROS-generating systems (e.g., O2 •−, H2O2, •OH) in the cochlea of guinea pigs (Tuncel et al. 2002). By comparing ROS level and measures of cochlear function, e.g., compound action potential (CAP) thresholds and changes in cochlear microphonic (CM) sensitivity, ROS generation has been shown to be an important pathway for lead-induced ototoxicity (Clerici and Yang 1996). Tetraethyl lead-exposed guinea pigs had significantly higher CAP thresholds in mid to high frequencies compared to controls. The lead acetate-exposed group also had higher (poorer) CAP thresholds than controls but less than the tetraethyl lead-exposed group. Pretreatment with α-phenyl-tert-butyl-nitrone, a free radical scavenger, significantly diminished tetraethyl lead-induced threshold shifts but not those induced by lead acetate. There were no differences in CM sensitivity between either of the lead groups and controls. These findings suggested that free radicals generated by lead exposure, especially tetraethyl lead, at least partially mediate lead toxicity in the inner ear (Tuncel et al. 2002). Importantly, they also indicate that the primary target for lead toxicity in the inner ear is not the sensory cells (little change in the CM) but the afferent fibers of the auditory nerve (reduced CAP).
Brainstem auditory evoked potential (BAEP, also known as brainstem auditory evoked response or auditory brainstem response [ABR]) provides a measure of nerve conduction velocity from the ears to the brainstem (Bhattacharyya 2013). BAEP waveform components represent the CAP in the distal portion of cranial nerve (wave I), second-order neuron activity beyond the cranial nerve in or near the cochlear nucleus (wave III), and activity in higher auditory structures (wave V). Interpeak latencies (i.e., I–III, III–V, or I–V) reflect changes in peripheral auditory nerve latency from changes in brainstem transmission in the auditory pathway.
In the occupational setting with lead-exposed workers, blood lead levels were associated with longer BAEP wave latencies (Bleecker et al. 2003) and higher auditory hearing thresholds (Chuang et al. 2007; Hwang et al. 2009). In a study conducted with 259 steel plant workers in Taiwan (mean blood lead level = 5.43 μg/dL) (Hwang et al. 2009), the odds ratios (ORs) of hearing loss (defined by threshold >25 dB HL) comparing people with blood lead levels ≥7 μg/dL with those with blood lead levels <4 μg/dL were 4.49 (3 kHz), 6.26 (4 kHz), 3.06 (6 kHz), and 6.16 (8 kHz). In a study of 30 lead-glazing Andean workers in Ecuador who had the mean blood lead levels of 45 μg/dL (standard deviation (SD) 19.5), there were dose-dependent associations between blood lead levels and delayed BAEP wave latencies (Counter and Buchanan 2002).
Two epidemiologic studies of the association between low-level lead exposure and hearing loss in the general population of adults have been reported (Choi et al. 2012; Park et al. 2010). In a study of 3,698 US adults aged 20–69 years using data from the National Health and Nutrition Examination Survey (NHANES) 1999–2004 (geometric mean blood lead = 1.54 μg/dL), individuals in the highest quintile of blood lead had 18.6 % (95 % confidence interval (CI), 7.4–31.1 %) higher hearing thresholds (pure-tone averages (PTA) at 0.5, 1, 2, and 4 kHz) than did those in the lowest quintile, with a significant trend across quintiles (p for trend < 0.001), after adjustment for sociodemographic and clinical risk factors and exposures to occupational and nonoccupational noise (Choi et al. 2012). Park et al. examined the associations between cumulative lead exposure measured by bone lead levels and hearing thresholds in 448 elderly community-dwelling men in Boston, Massachusetts, USA (Park et al. 2010). Patella bone lead levels were significantly associated with higher (poorer) hearing thresholds at 2–8 kHz, especially at the frequency of 4 kHz, and with higher odds of hearing loss (defined as PTA > 25 dB, OR = 1.48 (95 % CI, 1.14–1.91)), even after adjustment for important risk factors for hearing loss including occupational noise exposure. They also found a significant positive interaction between tibia bone lead and age in the longitudinal trajectory of hearing thresholds over up to 32 years of follow-up, suggesting that cumulative lead exposure may accelerate age-related hearing loss.
Several epidemiologic studies have also reported an association between lead exposure and hearing impairment among children. Two national surveys in the USA (NHANES II and Hispanic Health and Nutrition Examination Survey (HHANES)) examined children and adolescents (6–19 years of age) and found significant positive associations between blood lead and hearing thresholds at four frequencies (0.5, 1, 2, and 4 kHz) even at blood lead levels less than 10 mg/dL (Schwartz and Otto 1987, 1991). In a study conducted in an industrial area in Poland where 155 children aged 4–14 years with the median blood lead levels of 7.2 μg/dL were examined, blood lead levels were significantly associated with higher hearing thresholds at all frequencies from 0.5 to 8 kHz and with prolonged BAEP latency of wave I (Osman et al. 1999).
An interesting hypothesis was recently proposed. Lead poisoning is the primary cause of deafness of Ludwig van Beethoven (Stevens et al. 2013). The authors conducted an extensive review of the musical and medical literature and analyzed the information related to diagnoses for Beethoven’s hearing loss. They argued that evidence of the potential etiology proposed before including otosclerosis, autoimmune inflammatory bowel disease, mercury poisoning, and syphilis is lacking. After Beethoven’s mother died when he was 17 years old, he began to drink wine, particularly the adulterated or fortified Hungarian wine, and became alcohol dependent. It is well known that at that time lead was added illegally to sweeten wine, especially inexpensive wine. The authors concluded that Beethoven’s chronic consumption of wine tainted with lead is a better explanation of his hearing loss than other causes.
5.3 Cadmium
Cadmium is a trace element that has no essential function in humans. Cadmium, naturally found in the earth’s crust, is a by-product of the mining of zinc, lead, and copper ores. Cadmium is a serious soil contaminant from industrial waste and phosphate fertilizers, which eventually contaminates vegetables and fruit (ATSDR 2012). This makes most human foodstuffs a primary nonoccupational source of cadmium exposure within the general population (Satarug et al. 2010). Cigarette smoking is not only the major source of cadmium exposure among smokers but also an important cadmium exposure source even among nonsmokers due to secondhand smoke (Tellez-Plaza et al. 2012).
Various organ systems can be affected by cadmium exposure. A notable chronic high-dose effect is itai–itai (ouch–ouch) disease; cadmium poisoning occurred in the 1910s in Toyama Prefecture, Japan, characterized by softening of the bones and kidney failure (Emmerson 1970). Cadmium is a Group I carcinogen as determined by the International Agency for Research on Cancer (IARC) (Straif et al. 2009). Low-level chronic exposure has also been associated with kidney dysfunction, osteoporosis, cardiovascular disease, lower lung function, and diabetes (Satarug et al. 2010).
5.3.1 Cadmium and Oxidative Stress
Three potential mechanisms for cadmium-induced oxidative stress have been proposed (Cuypers et al. 2010): (1) induction of NADPH (nicotinamide adenine dinucleotide phosphate) oxidases, (2) replacement of redox-active metals, and (3) inhibition of antioxidant defense system including cadmium–thiol complexes formation.
NADPH oxidases generate superoxide (O2 •−) by transferring electrons derived from intracellular NADPH (Quinn et al. 2006). Superoxide can generally produce hydrogen peroxide (H2O2) spontaneously or through SOD. Despite its important function to kill bacteria and fungi, excess production may cause lipid peroxidation and oxidative damage. Cadmium exposure was found to upregulate NADPH oxidase gene expression in mouse kidneys (Thijssen et al. 2007) and hepatoma cell lines (Fotakis et al. 2005), which increases NADPH oxidase activity and in turn increases superoxide production. Another potential pathway is that cadmium replaces iron in various proteins and enzymes and, therefore, increases the free iron concentration (Dorta et al. 2003). Cadmium can mimic iron due to the same valency. Free redox-active metals like iron produce potent free radicals through the Fenton reaction followed by the Haber–Weiss reaction (Ercal et al. 2001; Jomova and Valko 2011).
Thiols play a critical role in cadmium-induced oxidative stress. Thiols, such as GSH and metallothionein, are known to detoxify cadmium by the formation of cadmium–thiol complexes, which reduces free cadmium and then blocks the aforementioned pathways leading to cadmium-induced oxidative stress (Thevenod 2009; Zalups and Ahmad 2003). Acute cadmium exposure has resulted in decreases in GSH and other antioxidants (e.g., SOD and CAT) and their activities (Casalino et al. 2002; Yalin et al. 2006). By contrast, chronic low-level exposure seems to activate those antioxidant enzymes, which in turn protect against cadmium-induced oxidative damage (Cuypers et al. 2010), a phenomena that mimics the “conditioning” effect of low-level noise, which similarly upregulates antioxidant defenses and decreases vulnerability of the inner ear to subsequent insult (Jacono et al. 1998). However, long-term persistent exposure to cadmium even at low levels may cause the antioxidant defense systems to fail, leading to an oxidative challenge with stress and aging (Ercal et al. 2001).
5.3.2 Cadmium and Ototoxicity
Ozcaglar et al. reported that cadmium can accumulate in ear ossicles and the labyrinth in rats (Ozcaglar et al. 2001). They also found that cadmium exposure at a concentration of 15 ppm CdCl2 caused defective hearing and kidney dysfunctions, but 5 ppm CdCl2 exposure caused only hearing loss without kidney dysfunction, suggesting that hair cells are more susceptible to cadmium than kidney tubule cells. Cadmium exposure increased the mean latency of ABR wave I but did not change wave III and V latencies. This result suggests that the cochlear component of hearing is more sensitive to cadmium toxicity than other, central, parts of the auditory system (Ozcaglar et al. 2001). Kim et al. examined cadmium-induced ototoxicity using auditory cell lines (HEI-OC1) and a mouse model (Kim et al. 2008, 2013). Cadmium exposure induced ROS generation, loss of mitochondrial membrane depolarization, the release of cytochrome c, activation of caspases, apoptosis, production of proinflammatory markers (interleukin (IL)-1β and IL-6), an increase of extracellular signal-regulated kinase activation in HEI-OC1 cells, and an elevation in ABR thresholds in mice. These adverse effects were significantly prevented by treatment with N-acetyl-l-cysteine (NAC), an antioxidant precursor to GSH (Dodd et al. 2008), and rosmarinic acid, a caffeic acid ester with antioxidant properties found in medicinal plants (Petersen and Simmonds 2003). They also observed that hair cells and Hensen cells were more responsive than Claudius cells to cadmium-induced apoptosis, which suggests that cadmium exposure may affect the basilar membrane vibration and sensory transduction and block nerve impulses transmitted to the brain via auditory nerves (Kim et al. 2008).
Two epidemiological studies reported the association between cadmium exposure and hearing loss. Shargorodsky et al. examined 875 adolescents aged 12–19 years who participated in the US NHANES 2005–2008 (Shargorodsky et al. 2011). After adjusting for important confounders, adolescents in the highest urinary cadmium quartile had a threefold higher odds of low-frequency hearing loss (defined as PTA at 0.5, 1, and 2 kHz > 15 dB) compared to the lowest quartile (odds ratio (OR) = 3.08 (95 % CI, 1.02–9.25)). Choi and colleagues investigated 3,698 US adults aged 20–69 years using data from the NHANES 1999–2004 (Choi et al. 2012). After adjusting for sociodemographic factors, occupational and nonoccupational noise exposures, and blood lead levels, the highest quintile group had a 74 % higher odds of hearing loss (defined as PTA at 0.5, 1, 2, and 4 kHz > 25 dB) compared to the lowest quintile group (OR = 1.74 (95 % CI, 1.12–2.70), with a significant trend across quintiles (p for trend = 0.01).
5.4 Mercury
Mercury is a transition metal that has several chemical forms. Metallic mercury (HgO) is a shiny, silver-white liquid used in thermometers, dental amalgams, and batteries (ATSDR 1999). Mercury can also form inorganic and organic compounds with salts (e.g., chlorine) or carbon that are typically exposed through ingestion. The most common and the most toxic form is methylmercury which can cross biological membranes, such as the blood–brain barrier and placenta, rendering it an important neurotoxicant (Aschner and Aschner 1990). Methylmercury is bioaccumulated in fish and seafood through the food chain, and thus, methylmercury concentrations are generally higher in large, long-lived fish (e.g., tilefish, shark, swordfish) and lower in small, and short-lived ones (e.g., salmon, pollock, catfish, shrimp) (ATSDR 1999). Metallic or inorganic forms of mercury through dental amalgam or occupational exposure (e.g., industrial use and gold mining) are also toxic to various degrees. Potential adverse effects of mercury exposure include neurological dysfunction, cardiovascular events, renal dysfunction, and dysfunction in endocrine, reproductive, and immune systems (Karagas et al. 2012; Rice et al. 2014). A notorious episode of severe mercury poisoning is Minamata disease, which occurred in the 1950s in Minamata Bay, Japan, where a chemical factory discharged large quantities of a mercury catalyst into the bay (Goldman 2007). Acute exposure to methylmercury caused paresthesia, ataxia, tremor, muscle spasticity, and visual and hearing impairment (Goldman 2007).
5.4.1 Mercury and Oxidative Stress
Mercury can induce oxidative stress through three potential mechanisms (Farina et al. 2011): (1) interaction with thiol and/or selenol (–SeH) groups from endogenous molecules, (2) disruption of mitochondrial electron transfer chain, and (3) an increase in extracellular glutamate levels and subsequent intracellular calcium influx and excitotoxicity.
Numerous studies have shown that both methylmercury and metallic mercury exposure can perturb the activities of proteins/enzymes containing thiol and/or selenol groups, such as GSH, GPx, Ca2+-ATPase, thioredoxin reductase, choline acetyltransferase, and enolase (Farina et al. 2013). Because selenols are more nucleophilic than thiols (Sugiura et al. 1976), mercury compounds have a higher affinity for selenols and hence preferentially bind to selenoproteins such as GPx and thioredoxin reductase (Branco et al. 2012; Farina et al. 2013). Thiol-containing proteins in mitochondria, such as respiratory chain complexes and mitochondrial creatine kinase, are also primary targets of methylmercury (Glaser et al. 2010), which can lead to mitochondrial collapse and mitochondrial oxidative damage (Franco et al. 2007). Methylmercury can also disrupt the complexes II (succinate dehydrogenase) and III (cytochrome bc1 complex)-mediated pathway in the mitochondrial electron transport chain, leading to the elevated H2O2 generation (Franco et al. 2007; Mori et al. 2007).
Disruption of extracellular glutamate homeostasis and subsequent calcium influx into neurons has been suggested as mercury-induced oxidative stress and neurotoxicity (Farina et al. 2011, 2013). Mercury compounds (e.g., methylmercury and inorganic mercury) lead to increased extracellular glutamate levels via the inhibition of astrocyte glutamate uptake (Aschner et al. 2000; Brookes and Kristt 1989) and the stimulation of glutamate release from presynaptic terminals (Albrecht and Matyja 1996; Reynolds and Racz 1987). Elevated extracellular glutamate can overactivate N-methyl-d-aspartate (NMDA)-type glutamate receptors, which results in calcium influx into postsynaptic neurons (Lafon-Cazal et al. 1993), leading to the activation of neuronal nitric oxide synthase (nNOS) and increased formation of nitric oxide (NO) (Himi et al. 1996; Yamashita et al. 1997), a reactive nitrogen species that can cause harmful oxidative and nitrosative stress responses (Thomas et al. 2008).
5.4.2 Mercury and Ototoxicity
Ototoxic effects of mercury compounds have been reported in animal models. Lin-Shiau and colleagues found that methylmercury and mercuric sulfide (HgS, also known as cinnabar, a naturally occurring HgS compound used as a sedative for more than 2,000 years in Asia (Kang-Yum and Oransky 1992)) were accumulated in the brainstem and caused increased hearing thresholds and prolonged absolute and interwave latencies of ABR in a mouse model (Chuu et al. 2001; Huang et al. 2008). They also observed suppressed Na+-/K+-ATPase activity, elevated NO levels, and lipid peroxidation in the brainstem in a dose-dependent manner. These results suggest that mercury-induced oxidative stress due to excess NO formation, lipid peroxidation, and altered Na+-/K+-ATPase activity in the brainstem could be an important pathway of mercury-induced ototoxicity. They also found that mercury exposure during the developmental periods (gestation and lactation) induced the mercury ototoxic effects listed above in offspring mice, suggesting that fetuses are vulnerable to mercury ototoxicity (Huang et al. 2011). Chronic low-level exposure to methylmercury (50 μg/kg/day) from birth to 7 years of age in monkeys caused high-frequency hearing loss at age 14 years, which also suggests the developmental period as a window of susceptibility to mercury ototoxicity (Rice and Gilbert 1992).
In human populations, high exposures to mercury compounds at workplaces or from contaminated community areas have been associated with adverse hearing outcomes. Auditory disturbances and hearing impairment were reported in Minamata disease patients (Harada 1995) and residents living near the Minamata Bay who have consumed fish and shellfish contaminated with low-level methylmercury (Ninomiya et al. 1995).
Different biomarkers reflect different mercury species exposures. Hair mercury mainly indicates methylmercury exposure, blood mercury indicates both methylmercury and elemental mercury exposure, while urinary mercury mainly indicates elemental mercury exposure (CDC 2013).
In a study of 26 mercury workers in Taiwan, Chang et al. reported prolonged wave III (5.73 ms vs. 5.80 ms) and IV (4.15 ms vs. 4.26 ms) latencies in the top one third (n = 10, hair mercury level = 53.9 (SD 34.6) μg/g) compared to the bottom one third (n = 11, hair mercury level = 4.9 (SD 1.1) μg/g) (Chang et al. 1995). In a study of 39 nonsmoking female dentists (age 40–45 years), there were significant positive associations between the number of amalgam fillings they had performed on patients and hearing thresholds at high frequencies (8, 11.2, 12.5, 14, and 16 kHz) (Rothwell and Boyd 2008). A cross-sectional study of 138 male workers of a fluorescent lamp factory in Egypt reported that individuals with hearing impairment had higher urinary mercury levels (51.4 (SD 19.5) μg/g creatinine) than those without hearing impairment (42.3 (SD 16.6) μg/g creatinine) (Al-Batanony et al. 2013).
People who work at gold mining areas are at high risk of mercury poisoning which can occur through inhalation of elemental mercury vapor during amalgam burning in the gold extraction process and/or ingestion of methylmercury from contaminated fish. Counter and colleagues examined Andean children and adults living in gold mining areas of Ecuador (Counter et al. 1998, 2012; Counter 2003). In children whose ages were between 4 and 14 years (sample sizes ranged from 22 to 31), blood mercury levels ranged from 2 to 89 μg/L with medians of 7–23 μg/L, which are much higher than the median blood mercury level of 0.26 μg/L reported for children in the USA (Schober et al. 2003). There were significant positive correlations between blood mercury levels and the absolute latency of wave V (r = 0.38, p = 0.03) and the I–V interwave interval (r = 0.41, p = 0.02) (Counter 2003). They also observed significant positive correlations between blood mercury and hearing thresholds (Counter et al. 1998) and acoustic stapedius reflex thresholds (Counter et al. 2012) in children. In the same studies (Counter et al. 1998, 2012), they also examined adult gold mining workers who had blood mercury levels between 2 and 32 μg/L with a median of 6 μg/L which is also higher than that found in the US general population (geometric mean of 1.03 μg/L) (Park et al. 2013). However, surprisingly, no significant associations between blood mercury and hearing outcomes were found in adults.
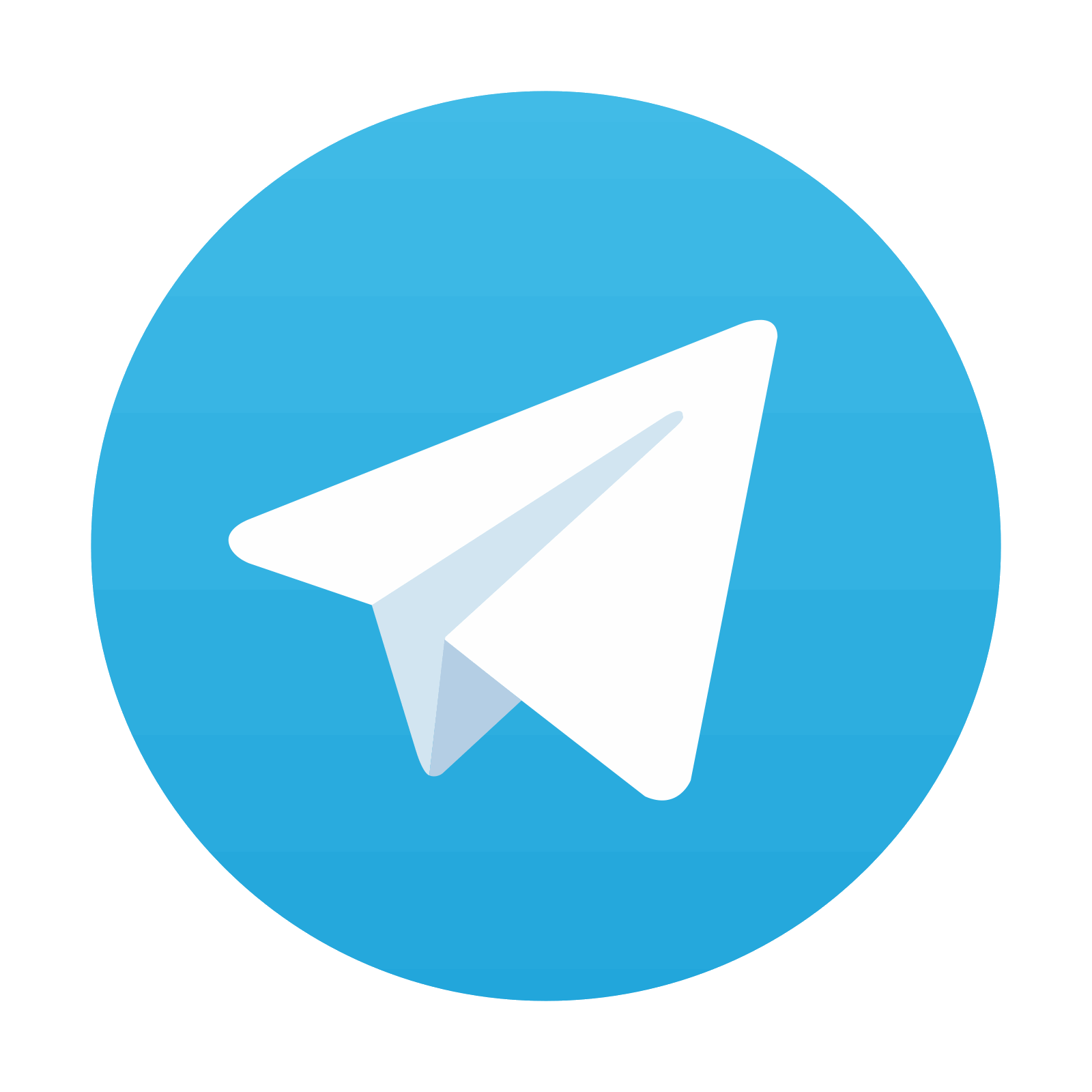
Stay updated, free articles. Join our Telegram channel
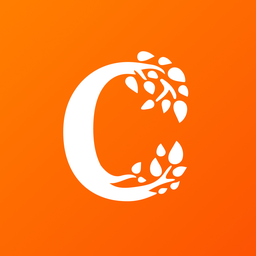
Full access? Get Clinical Tree
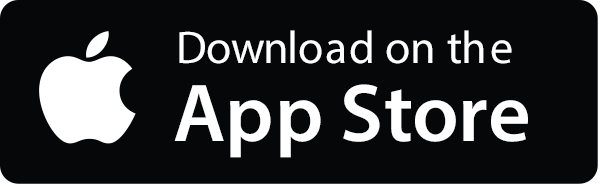
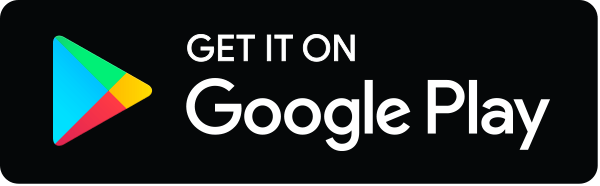