Chapter 5 Renal physiology
5.1 Body fluids and their distribution
Water accounts for 60% of body mass in males, i.e., over 40 L in a 70 kg adult. This fraction is somewhat lower in females, at about 50%, because of the increased average body content of fatty tissue, which contains less water than other tissue types. Total body water is divided between intracellular (two-thirds of total body fluid) and extracellular compartments (one-third of total body fluid), with plasma forming a major subcompartment (one-quarter of extracellular fluid) within the extracellular space (Section 1.1, Fig. 2). Water can move freely between these fluid spaces and this exchange is controlled by pressure gradients across the cell membrane (which separates intracellular and extracellular spaces) and the capillary wall (which separates plasma from interstitial fluid). In each case, the distribution of fluid depends on the net effect of hydrostatic and osmotic forces. The solutes controlling osmosis at the two sites, however, are quite different. Only those species which cannot diffuse from one compartment to another will exert an osmotic effect (Section 1.3) and the relevant barriers to diffusion demonstrate very different solute permeabilities.
Proteins
Ions diffuse rapidly across the capillary wall but proteins do not, so the osmotic pressure gradient affecting distribution of fluid between plasma and other, extravascular spaces within the extracellular compartment depends on the transcapillary gradient in protein concentration. It should be remembered, though, that protein only accounts for a tiny fraction (<1%) of total plasma osmolality (Section 3.8).
Ionic composition of extracellular fluid
The extracellular concentrations of a number of important ions are commonly determined using plasma from venous blood samples. Normal values are summarized in Table 8. Although the total charge carried by all the cations and anions must be equal, a significant fraction of the anion load is not accounted for in routine measurements. It can be seen that the sum of the measured cations exceeds the sum of the measured anions, i.e.,
Table 8 Typical plasma electrolyte values in a sample of venous blood from a normal individual. (Note: the contribution of ions labelled below as ‘others’ is not routinely measured.)
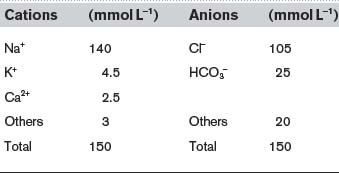
The difference between these totals is called the anion gap (17 mmol L−1 in this case). This represents the contribution made by anions not normally measured in the clinical laboratory, e.g., sulphate, phosphate, proteins and other organic anions. Any condition which generates additional anions of this sort, e.g., the anions released by dissociation of ketoacids in diabetes mellitus (Section 8.7), will increase the anion gap.
5.2 Relevant renal structure
There are two kidneys, each with an outer cortex and inner medulla (Fig. 78A). Urine is formed within functional subunits known as nephrons (Fig. 78B). Each nephron contains a glomerulus, consisting of a tuft of capillaries, along with the afferent and efferent arterioles through which blood enters and leaves (Fig. 78C). The glomerulus is surrounded by the epithelium of the Bowman’s capsule and these structures combine to form the renal corpuscle. This is the site of initial plasma filtration. The resulting filtrate is then modified by a variety of secretory and reabsorptive processes as it passes in turn through the proximal convoluted tubule, the loop of Henle, the distal convoluted tubule and the collecting duct (Fig. 78B). The glomeruli and convoluted tubules lie within the renal cortex while the loops of Henle and collecting ducts extend down into the medullary region. The end product, urine, is eventually delivered via the renal pelvis to the ureter, which empties into the bladder.
Ultrastructural features of the nephron
The walls of each nephron are made up of a single layer of epithelial cells, although the appearance of these cells is not uniform throughout. Specialized epithelial cells in Bowman’s capsule called podocytes surround the glomerular capillaries and contribute to the filtration barrier (Section 5.3, Fig. 79). The cells lining the convoluted tubules carry a brush border of microvilli on their luminal surface and contain large numbers of intracellular mitochondria. These features are particularly pronounced in the proximal convoluted tubule and provide a large surface area and rich supplies of ATP, appropriate features for a region specializing in active transport. In the descending limb of the loop of Henle, by contrast, the epithelium is flat, with few organelles. This is consistent with a low level of active transport and high permeability to fluid and electrolytes. Cells in the more distal, thick portion of the ascending limb, however, are more like those in the tubules. These cells are involved in active ion transport and show a very low level of permeability to water, probably because of well-developed tight junctions between adjacent epithelial cells in this area.
Blood supply to the nephron
The blood supply to the kidney is delivered via the renal artery. The glomerular capillaries are supplied through an afferent arteriole but drain through an efferent arteriole rather than a venule (Fig. 78C). Blood from the efferent arteriole enters the peritubular capillary beds and the vasa recta. The latter vessels descend into the medulla to supply the loops of Henle and collecting ducts. Eventually all these vessels drain through the cortex into the renal veins.
5.3 Glomerular filtration
The initial step in the formation of urine is production of a plasma filtrate within the glomerulus. As is the case for capillary exchange elsewhere in the body (Section 3.8), the driving forces involved are the hydrostatic and osmotic pressure gradients between the glomerular capillaries and Bowman’s space (Fig. 79). Glomerular filtration differs from filtration in general systemic capillary beds, however, in that these gradients result in the efflux of much larger volumes of fluid, with a total filtration rate of approximately 120 ml min−1 for both kidneys. This reflects structural specializations in the glomerulus which favour filtration.
High glomerular filtration coefficient
Forces controlling filtration
The balance between filtration (hydrostatic) and absorption (colloid osmotic) forces in the glomerulus differs from that in other capillaries (Section 3.8).
Hydrostatic pressures
The hydrostatic pressure within glomerular capillaries is higher than elsewhere (about 50 mmHg). This is largely caused by the high outflow resistance offered by the efferent arteriole, which raises the upstream pressure. At the same time, there is very little decline in pressure between the afferent and efferent arterioles as a result of the comparatively low resistance of the glomerular capillaries (Pressure fall = Flow × Resistance; Section 3.6). Since the pressure within Bowman’s space is about 10 mmHg, there is a net hydrostatic pressure gradient of 40 mmHg favouring glomerular filtration along the entire length of the glomerular capillary.
Osmotic pressure
Because proteins cannot cross the glomerular wall they exert a colloid osmotic pressure opposing filtration, just as they do in other capillaries (Section 3.8). At the afferent arteriolar end of the glomerulus this equals the normal plasma colloid osmotic pressure of about 25 mmHg, so there is a net filtration gradient (hydrostatic gradient – osmotic gradient) of about 15 mmHg at that point. This is not dissimilar to the gradient at the arteriolar end of a normal capillary, but in the glomerulus this results in a much faster rate of filtration because of the very high hydraulic conductivity of the capillary wall. As a result of this fluid removal, the concentration of the plasma proteins rises and this increases the osmotic gradient opposing filtration. Eventually the osmotic gradient exactly matches the hydrostatic gradient so no further filtration occurs.
Interaction of forces in the glomerulus
The values of the relevant forces at different points along the length of the glomerular capillary can be plotted graphically to indicate the region over which filtration will occur (Fig. 80). It should be noted that the osmotic gradient rises to balance the hydrostatic gradient exactly but never exceeds it, so there is no absorption of fluid at any point along the glomerular capillaries.
Regulation of glomerular filtration rate and renal blood flow
The glomerular filtration rate is the total rate of renal filtration in both kidneys and normally equals about 120 ml min−1. This is remarkably constant over a wide range of conditions. Physiological control of filtration is a complex and poorly understood subject, but glomerular filtration rate will be increased by factors which increase any of the following variables:
The maintenance of renal blood flow is particularly important for normal filtration, since this is the main determinant of glomerular capillary pressure and flow. Renal blood flow is high (about 1.2 L min−1) and shows remarkably little variation over a wide range of arterial pressures, providing us with one of the best examples of autoregulation of local blood flow (Section 3.7, Fig. 50). Therefore, if arterial pressure falls, dilatation of the afferent arterioles reduces renal vascular resistance and so helps to limit the decrease in blood flow. At very low pressures (e.g., mean arterial pressure < 80 mmHg), autoregulation breaks down and renal blood flow declines more rapidly. Reflex stimulation of sympathetic nerves to the kidney under these conditions may further reduce both glomerular blood flow and hydrostatic pressure by constricting the afferent arterioles. Therefore, both glomerular filtration and urine production may be dramatically reduced in surgical shock with marked arterial hypotension.
5.4 Modification of glomerular filtrate
Mechanisms leading to reabsorption of Na+ and water
Proximal convoluted tubule
Renal transport of Na+ and water are closely linked. The mechanisms involved are particularly important since more than 170 L of fluid is filtered out of the plasma each day in the glomeruli, and the vast bulk of this has to be rapidly reabsorbed to avoid fatal depletion of the extracellular fluid. Most of this reabsorption depends on tubular cells, which are specialized for active transport. Energy released by the breakdown of ATP drives Na+ across the tubular epithelium into the peritubular fluid. Chloride ions follow passively through electrostatic attraction and the resulting osmotic gradient draws water out of the tubule (Fig. 81). The net effect is reabsorption of a NaCl solution essentially isosmotic with plasma. As a result, the volume of the filtrate is decreased but its osmolality does not change.
About 70% of the filtered Na+ is automatically reabsorbed from the proximal convoluted tubule in this way. This fraction remains relatively fixed across a wide range of Na+ filtration rates, i.e., a fixed fraction of the total quantity of Na+ presented to the transport system is reabsorbed (Fig. 82). Renal transport processes of this kind are referred to as gradient–time limited systems.
Loop of Henle
In the loop of Henle active reabsorption of Na+ leads to passive Cl− transport, but this is confined to the thick portion of the ascending limb (Fig. 81). Unlike the convoluted tubules, however, this region is impermeable to water and so ion transport raises the concentration of NaCl and, therefore, the osmolality in the medullary fluid around the whole of the loop of Henle. The thin descending limb is permeable both to ions and water, so as the fluid from the proximal convoluted tubule descends through the medulla it is both concentrated and reduced in volume by the passive influx of Na+ and Cl− and the osmotic removal of water. This concentrated solution becomes more dilute as it ascends the opposite limb of the loop of Henle because of the active removal of solute, so that the solution entering the distal convoluted tubule actually has a lower osmolality (about 100 mosmol kg−1) than that leaving the proximal tubule (300 mosmol kg−1). This mechanism allows for the production of hypo- as well as hypertonic urine.
The most important effect of ion transport by the loop of Henle is to increase the osmolality of the interstitial fluid in the medulla of the kidney, which rises to a maximum of about 1200 mosmol kg−1 at the bottom of the loop (Fig. 81). This provides the driving force for subsequent reabsorption of water from the collecting ducts.
Distal convoluted tubule and collecting ducts
There are two important mechanisms which play a role in Na+ and water reabsorption in these regions.
Sodium reabsorption
Most of the remaining Na+ load is actively reabsorbed from the distal convoluted tubule and collecting ducts so that less than 1% of the filtered Na+ is excreted in the urine. These transport processes are analogous with those in the proximal convoluted tubule, with isosmotic reabsorption of NaCl and water. A small but important fraction of this resorption depends on stimulation by the hormone aldosterone, allowing Na+ excretion to be regulated so as to maintain a normal extracellular fluid volume.
Water reabsorption
The low osmolality of the fluid entering the distal convoluted tubule allows for osmotic reabsorption of water into the extracellular fluid of the cortex. Further osmotically driven water absorption occurs from the collecting ducts as they descend through the medulla towards the renal pelvis (Fig. 81). This depends on the high osmolality generated in the surrounding medullary interstitium by the ionic pumps of the loop of Henle. Since this water reabsorption occurs without any parallel ion transport, the volume of the urine is decreased and its osmolality is increased. The distal convoluted tubule and collecting duct remain relatively impermeable to water, however, unless stimulated by circulating antidiuretic hormone (ADH), and a large volume of urine (a diuresis) with a low osmolality is produced in the absence of this hormone. With maximal ADH secretion, a small volume of highly concentrated urine is excreted (Fig. 81). This provides an endocrine control mechanism which can rapidly alter renal water absorption so as to correct abnormalities of extracellular fluid volume or osmolality.
The countercurrent multiplier mechanism in the loop of Henle
Let us assume that the system is initially filled with isotonic solution (300 mosmol kg−1) from the proximal convoluted tubules. The ion pumps will then transfer solute from the ascending limb into the interstitium until the concentration gradient generated across the epithelium exactly opposes the energy available from the active transport system (Section 1.3). In a simple, single conduit system, this might limit the gradient to about 200 mosmol kg−1, with a maximal interstitial osmolality of about 400 mosmol kg−1 (Fig. 83A). In the countercurrent system, however, the solution in the permeable descending limb equilibrates with the interstitium and so becomes concentrated. As more (isotonic) fluid enters at the top of the descending limb, concentrated fluid is forced round into the ascending limb, reducing the transepithelial osmotic gradient while maintaining a high total interstitial osmolality (Fig. 83B). Ion pumping can proceed once more, until a 200 mosmol kg−1 gradient is again achieved. In this example, the osmolality in the medullary interstitium and lower part of the descending limb is raised to 500 mosmol kg−1 as a result. Repeated cycles of ion pumping followed by fluid transfer can thus generate a higher medullary osmolality than would otherwise be possible. At the same time, a gradient in osmolality develops along the length of the loop of Henle with low values in the upper medulla and high values adjacent to its tip.
Countercurrent exchange in the vasa recta
The blood vessels supplying the medullary regions of the kidney (the vasa recta) are arranged in a U configuration so that blood descends one limb before ascending back to the cortex (Fig. 78C). As blood descends through areas of increasing osmolality, NaCl diffuses across the capillary walls, increasing plasma osmolality (Fig. 84). If these vessels drained into the venous system directly from the medulla, they would leach away a great deal of solute, increasing the energy needed to maintain the high medullary osmolality. As the hypertonic plasma ascends through the medulla again, however, ions diffuse back into the surrounding interstitium. The net effect is the continuous recycling of fluid and solutes as blood flows in opposite directions through the parallel limbs of the vasa recta, a process known as countercurrent exchange.
Regulation of renal Na+ and water reabsorption
Three hormones play an important role in the regulation of extracellular fluid volume and osmolality through their actions on renal reabsorption of Na+ and water (Fig. 85).
Antidiuretic hormone
This peptide hormone is manufactured by cells in the supraoptic and paraventricular nuclei of the hypothalamus and is released from the posterior pituitary (Section 8.2). Antidiuretic hormone (ADH) secretion is stimulated by:
Antidiuretic hormone increases the water permeability in the collecting ducts and the distal convoluted tubule by stimulating the insertion of water-selective channels in epithelial cell membranes. These channels, known as aquaporins, promote reabsorption of water due to the osmotic gradients across the walls of the distal convoluted tubule (low intratubular osmolality) and the collecting ducts (high medullary interstitial osmolality). As a result, the extracellular fluid volume is expanded while its osmolality is reduced. Antidiuretic hormone also helps to elevate arterial blood pressure by direct vasoconstriction, leading to its alternative name of vasopressin (Section 3.6).
The renin–angiotensin–aldosterone system
All of these stimuli are particularly relevant with regard to regulation of extracellular fluid volume, since this directly controls plasma volume and so affects blood pressure (Section 3.6). In each case changes are detected by the juxtaglomerular apparatus, a structure formed by the close association of specialized juxtaglomerular cells in the wall of the afferent arteriole and the macula densa in the distal convoluted tubule. Renin is released from the juxtaglomerular cells and catalyzes the conversion of the plasma precursor protein angiotensinogen into angiotensin I. This is further converted to angiotensin II by angiotensin converting enzyme on the surface of vascular endothelium. Angiotensin II promotes release of the steroid hormone aldosterone from the adrenal cortex (Section 8.5) and this in turn stimulates reabsorption of Na+ and water from the distal convoluted tubule and collecting ducts, leading to expansion of the extracellular space and increasing blood pressure. These effects take several hours to develop fully. Although aldosterone only influences the reabsorption of the final 2–3% of the total load of filtered Na+, this represents a volume of up to 5 L of isotonic fluid in 24 hours and so provides an important mechanism for the control of extracellular volume. It should also be noted that high levels of aldosterone favour renal conservation of fluid by reducing urine volume but have limited effects on plasma or urine osmolality.
Angiotensin II has a variety of other actions with complementary effects, for example:
Transport of K+
Potassium ions are reabsorbed and secreted in different parts of the nephron. Active reabsorption from both the proximal convoluted tubule and the ascending limb of the loop of Henle reduces the K+ load to less than 10% of the filtered K+. It is the rate of K+ secretion in the distal convoluted tubule, however, which largely determines the rate of K+ excretion in the urine. Aldosterone stimulates this secretion and is the most important regulator of plasma [K+] since adrenocortical secretion of aldosterone is directly stimulated by K+ (Section 8.5).
Reabsorption of Ca2+ and phosphate ions
Both these ions are actively reabsorbed from the tubular fluid, particularly in the proximal convoluted tubule, so that normally only about one-third of the filtered load reaches the urine. Reabsorption is hormonally controlled by parathormone, which promotes Ca2+ reabsorption while inhibiting that of phosphate. This is an important mechanism in the regulation of plasma Ca2+ concentrations (Section 8.4).
Transport of H+ and HCO3−
H+ secretion and HCO3 − absorption
Bicarbonate is one of the most important pH buffers in the body (Section 5.9). Since it is freely filtered in the glomerulus it is vital that the renal tubules should have an efficient mechanism for HCO3 − absorption, otherwise its loss in the urine would quickly lead to fatal acidosis. Protons and HCO3 − are generated within the tubular epithelial cells by dissociation of carbonic acid formed by the reaction of CO2 with water (Fig. 86A). This reaction is catalyzed by the enzyme carbonic anhydrase. The H+ is actively transported into the tubular lumen while the HCO3 − diffuses into the peritubular capillaries, maintaining electrical neutrality inside the cell. Secreted H+ has to be buffered in the urine to limit the [H+] gradient between the tubular cells and the lumen, otherwise it would quickly become too large for the active transport system, preventing further secretion. Filtered HCO3 − reacts with H+ to form CO2 and H2O in the tubular fluid. Carbon dioxide can diffuse freely back across the tubular cell membrane, completing the cycle. The net effect is that one HCO3 − ion is absorbed into the peritubular plasma from the filtrate for each H+ which is secreted.
This mechanism conserves HCO3 − and, under normal conditions, all the filtered HCO3 − is absorbed (mainly in the proximal convoluted tubule) and none is excreted in urine. If the rate at which HCO3 − is filtered exceeds the rate of H+ secretion, however, then the excess cannot be absorbed and will be excreted, making the urine alkaline. This mechanism tends to reduce plasma HCO3 − during alkalosis, and thus tends to lower the pH (see Eq. 41 in Section 5.9).
H+ excretion and HCO3 − generation
Although the mechanisms described above couple H+ secretion to HCO3 − absorption, they do not lead to any net excretion of H+ in the urine since the CO2 produced diffuses back out of the tubular lumen (Fig. 86A). This underlines the point that renal secretion (a tubular cellular mechanism) and excretion (permanent removal in the urine) are not the same thing. Renal excretion of acid still depends on tubular secretion but in this case the H+ is buffered by phosphate species such as HPO4 2−, or ammonia (NH3). The H2PO4 − and ammonium (NH4 +) ions formed cannot cross the lipid membrane of the tubular epithelium and are excreted in the urine (Fig. 86B, C). Phosphate enters the lumen by filtration but the NH3 is actually secreted by the tubular cells, much of it having been synthesized from glutamine within the cell (ammoniagenesis).
One important feature of H+ buffering by phosphate and ammonia is that it leaves the HCO3 − produced by dissociation of carbonic acid within the tubular cells to diffuse into the extracellular fluid. Since this amounts to generation of additional HCO3 − over and above that which was filtered in the glomerulus, it can actually raise plasma [HCO3 −], rather than simply preventing it from decreasing as absorption of filtered HCO3 − does. Bicarbonate is an important base within the body, and this mechanism provides for renal compensation of a respiratory acidosis. For example, during a chronic respiratory acidosis both the rate of H+ secretion and the rate of NH3 production are upregulated within the first week, leading to an increase in plasma [HCO3 −]. This tends to reverse the fall in blood pH, at least partially compensating for the original defect (Sections 4.4 and 5.9).
Reabsorption of glucose and other nutrients
Glucose is freely filtered in the glomerulus so the concentration in the filtrate equals that in plasma. This means that the glucose load presented to the tubules is proportional to the plasma glucose concentration (Fig. 87). Normally, the rate of active glucose reabsorption in the proximal convoluted tubule equals the rate of filtration, so no glucose appears in the urine. This reuptake can eventually be saturated, however, and the maximum achievable rate of glucose reabsorption is known as the transport maximum (Tmax). If the rate of glucose filtration rises above Tmax, the excess load will all be excreted in the urine (Fig. 87). This occurs when the plasma glucose concentration rises above about 11 mmol L−1, so this is regarded as the renal threshold concentration, above which glucose will be excreted in the urine (glycosuria). Glycosuria is an important feature of diabetes mellitus, in which a deficiency of the hormone insulin leads to abnormally high glucose concentrations (Section 8.7).
The relationship between plasma glucose concentration and urinary excretion rate is curved over a range of concentrations immediately above threshold. This region of splay reflects small variations in the maximum transport rate for different tubules within the kidneys. Eventually all glucose transport systems are saturated, and urinary excretion then rises linearly with plasma glucose since any increase in the filtered glucose load now results in an equal increase in glucose excretion.
Tubular maximum transport mechanisms of this sort are also involved in the reabsorption of other filtered nutrients from the proximal convoluted tubule, especially amino acids. Systems of this kind should be contrasted with the gradient–time dependent resorption of Na+, in which a fixed fraction of the filtered load is reabsorbed (Fig. 82).
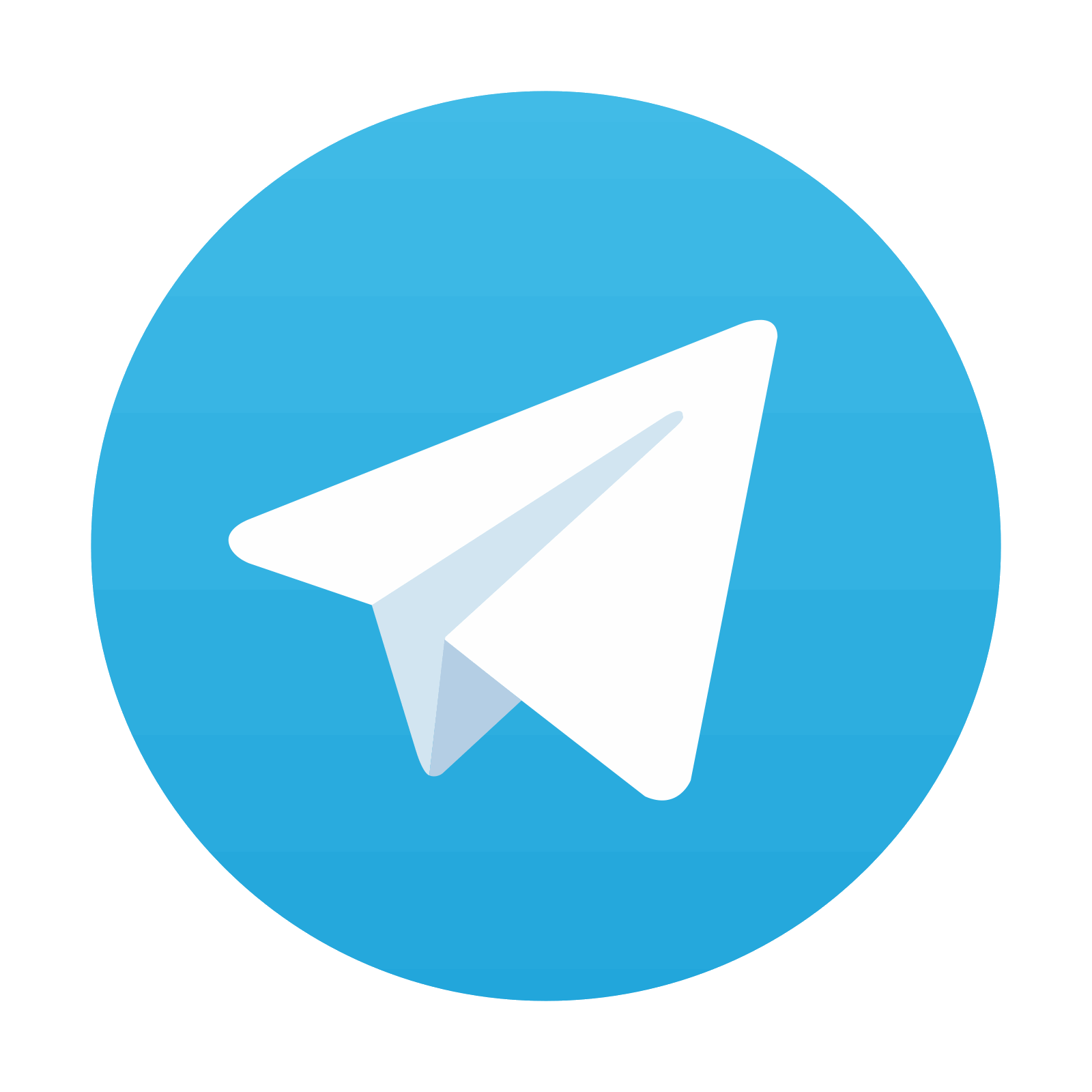
Stay updated, free articles. Join our Telegram channel
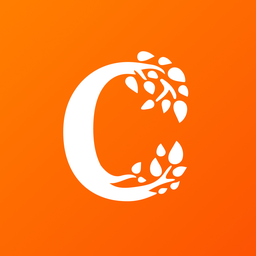
Full access? Get Clinical Tree
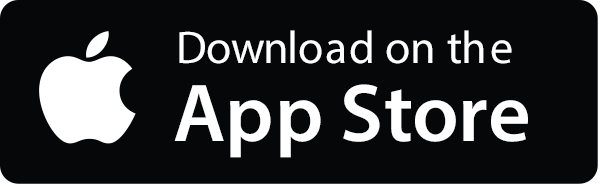
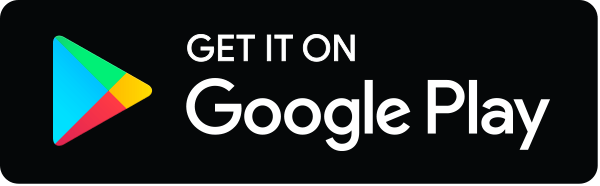