Fig. 1
TNF receptor signaling regulation of cell fate. Upon the binding of TNF to its receptor TNFR1, RIPK1 is recruited to TNFR1 and is subsequently ubiquitinated. The polyubiquitinated RIPK1, in turn, binds to NEMO, the regulatory subunit of NF-κB, to promote NF-κB activation, which leads to the induction of pro-survival genes to counter the death signals. Cell survival is a result of this pathway. The polyubiquitinated RIPK1 can also migrate to the cytoplasm, where RIP1 is de-ubiquitinated by A20, the de-ubiquitylating enzyme. RIPK1 and RIPK3 can then form a pro-necrotic complex followed by phosphorylation on both kinases and induction of necroptosis. In circumstances in which caspase-8 is activated, RIPK1 and RIPK3 can be cleaved by caspase-8, and the pro-necrotic complex is blunted, which stimulates the cell to undergo apoptosis
The pseudokinase mixed lineage kinase domain-like (MLKL) protein is a substrate of RIPK3 and required for necroptosis [6, 19]. Unlike its previously discovered function in regulating mitochondrial fission, MLKL recruitment and phosphorylation caused by RIP homotypic interaction motif (RHIM)-dependent oligomerization and intramolecular RIPK3 autophosphorylation [20, 21] results in an activated state able to induce necroptosis [22]. Furthermore, several studies have deciphered a role for MLKL in necroptosis. MLKL oligomerization induced by RIPK3 and plasma membrane localization is associated with its cytotoxicity [23–26]. MLKL binds to phosphatidylinositol phosphates (PIPs) [23, 25] and subsequently modifies sodium or calcium influx through ion channels, thereby increasing osmotic pressure and promoting plasma membrane rupture [24, 26, 27].
It is unclear what the mechanism in which the necrosome causes cell death is. Necroptosis and necrosis shares several identical sub-cellular events, including: mitochondrial membrane hyperpolarization, oxidative burst, and lysosomal and plasma membrane permeabilization. However, the underlying mechanisms for these processes may differ [28]. Reactive oxygen species (ROS) potentially lead to cell death by directly oxidizing or triggering various downstream pathways in the mitochondria [29–31]. RIPK3 accelerates mitochondrial ROS production and mitochondrial metabolism through the activation of a series of metabolism-related enzymes, including nicotinamide adenine dinucleotide phosphate (NADPH) and c-Jun N-terminal kinases (JNK) [32, 33]. Through an ADP/ATP-related pathway in addition to ROS production, mitochondria affect necrotic cell death. Adenine nucleotide translocase (ANT), an ADP/ATP carrier located in the inner mitochondrial membrane, is required for the synthesis of ATP in the mitochondria. RIPK1-dependent inhibition of ANT is reportedly involved in the programmed necrosis induced by TNF-α and zVAD-fmk, whereas the latter potentially blocks the ability of ANT to transport cytoplasmic ADP and thereby induces massive ATP depletion in mitochondria. The activity of ANT is potentially affected by interactions with VDAC and cyclophilin D (CYPD). Two other potential executional proteins are calcium-dependent phospholipase A2 (cPLA2) and lipoxygenase (LOXs). cPLA2 plays an important role in TNF-α-induced necrotic cell death in L929 cells and MEFs [34]. LOXs act as downstream effectors of cPLA2 and lead to the disruption of organelle and plasma membranes [35]. LOXs is reportedly involved in both apoptosis and necrosis induced by TNF-α, although the exact mechanism has yet to be defined [36, 37].
Necroptosis can initiate inflammation. The triggering of inflammation by necroptosis has been seen in a study using mice with deletion of FADD [38] or Casp8 [39] in intestinal epithelial cells (IECs). In this study, it was observed that RIPK3-dependent cell death caused intestinal inflammation. RIPK3-mediated necroptosis may play a role in the pathogenesis of Crohn’s disease, as evidenced by the high RIPK3 expression in Paneth cells of these patients [39]. Necroptosis has been found to stimulate the immune system to elicit inflammatory responses and has also been characterized in animal models of acute pancreatitis, ischemic injury, and neurodegeneration [40–43]. RIPK3−/− mice are protected from systemic inflammation caused by TNF stimulation and experimental sepsis induced by cecal ligation and puncture (CLP) [44, 45]. RIPK1 and RIPK3 also play crucial roles in the pathogenesis of Salmonella enterica serovar and S. typhimurium infection [46]. Necrotic macrophages have been observed in atherosclerosis lesions from both animals and human patients [47]. RIPK3-dependent necroptosis is a key driver of inflammation in atherosclerosis; RIP3 deficiency alleviates macrophage necrosis in advanced atherosclerosis lesions in atherosclerosis-prone LDL-R−/− or ApoE−/− mice [48]. The contribution of RIPK1-dependent necroptosis to multiple organ failure has also been observed in models of ischemia reperfusion (IR) and can be rescued by Nec-1 inhibitor [49–51]. In addition, necroptosis has been shown to contribute to neuronal damage in neonatal brain injury [52].
Necrosis and necroptosis both influence host disease outcomes through triggering inflammation. Determining the relative contribution of necroptosis-dependent and -independent pathways in inflammation may lead to new and more specific therapeutic targets.
3 Apoptosis and Inflammation
Apoptosis is a major type of cell death. Two separate signaling cascades for apoptosis have been identified: intrinsic and extrinsic pathways [53]. The binding of Fas plasma membrane death receptor to Fas ligand (Fas-L) or other like receptors triggers the extrinsic pathway [54]. Fas-L combines with Fas to form a death complex. The Fas/Fas-L composite binds with pro-caspase-8 and a death domain containing protein (FADD) to form the death-inducing signaling complex (DISC). The protein complex then activates pro-caspase-8 which then activates pro-caspase-3 [55]. Mitochondrial pro-enzymes control the intrinsic pathway. Stimuli affecting the cell causes outer mitochondrial membranes to become permeable and release cytochrome c into the cytosol In the cytosol, cytochrome c binds with Apaf-1, an adaptor protein, and forms the apoptosome, triggering downstream caspase-9 [56]. Caspases -3 and -7, processed by caspases-8, -9, and -10 are executioner caspases that cleave many substrates, resulting in apoptosis. The biochemical and morphological changes caused by these caspases include membrane blebbing, nuclear condensation, phosphatidylserine exposure, and genomic DNA fragmentation.
Inflammation and apoptosis are heavily related as the onset of inflammation activates a number of signaling pathways that are critical in the regulation of apoptosis. Absent in melanoma 2 (AIM2), a member of the pattern recognition receptors (PRRs) in the cytoplasm, has been found to activate caspase-3 in parallel with caspase-1 [57]. AIM2 can recognize DNA released by the cytosolic bacteria [58], whereas NLRP3, another member of the cytoplasmic PRRs, responds to the bacterial pore-forming toxin nigericin [59], both of which elicit apoptotic caspase activation [60, 61]. Apoptotic responses can be observed in wild type cells responding to AIM2 or NLRP3 stimuli [59]. AIM2 and NLRP3 inflammasome -dependent apoptosis requires caspase-8, which is recruited to the inflammasome through interaction between its DED domains and the pyrin domain (PYD) of apoptosis-associated speck-like protein containing a caspase activation and recruitment domains (CARD), an adaptor molecule of the inflammasome [58, 59, 62]. In contrast, BCL-2 can negatively regulate NLRP3 inflammasome activation by preventing the cytosolic release of mitochondrial DNA [63].
In order to initiate phagocytosis of apoptotic cells, these cells release signals that are composed of either newly expressed molecules or modified existing molecules [64]. Phagocytosis of apoptotic cells is an anti-inflammatory mechanism. Phosphatidyl serine (PS) localized to the outer leaflet of the plasma membrane is the predominant “eat me” molecule upon apoptosis [64, 65]. Specific molecules such as milk fat globule epidermal growth factor 8 (MFG-E8) links PS to phagocyte avb3 integrin [64], whereas growth-arrest-specific 6 (GAS6) links PS to the receptor tyrosine kinase MER [64]. PS acts as a ligand for the T-cell immunoglobulin domain and mucin domain (TIM)-4 molecule on macrophages and dendritic cells (DC) [66], and TIM-4 helps promote the uptake of apoptotic cells [67]. Two other molecules, brain-specific angiogenesis inhibitor 1 (BAI1) and stabilin-2, have also been shown to mediate uptake of apoptotic cells via recognition of PS [68, 69].
Although apoptotic cells are rarely seen under normal physiological conditions, the build-up of uncleared apoptotic cells is an indicator of many distinct diseases and the expression of inflammation and infection Tissue-resident cells, as a response to infection or tissue injury, detect PAMPs and DAMPs. Leukocytes then collect at the site of inflammation. Here, innate immune cells, such as neutrophils, are usually first to appear then macrophages and mononuclear cells appear afterwards [70]. This initial robust immune response is designed to destroy invading pathogens and enhance tissue repair [71, 72]. After the initial threat is eliminated, leukocytes are cleared. Leukocytes are primarily cleared through neutrophil apoptosis and phagocytosis [73, 74]; however, another route of clearance is transepithelial migration into the airway lumen in regards to lung inflammation [75] or via lymphatic vessels [76]. The phagocytosis of pathogens, such as Escherichia coli or Staphylococcus aureus, promotes neutrophil apoptosis following neutrophil recruitment, which is termed phagocytosis-induced cell death (PICD) [77]. This response is believed to be primarily protective for the host, and incidentally, pharmacological acceleration of neutrophil apoptosis is protective in pneumococcal meningitis by reducing the incidence of brain hemorrhage [78]. The failed clearance of apoptotic neutrophils can lead to a prolonged inflammatory response , and this phenomenon has been observed in disease, including chronic obstructive pulmonary disease (COPD) [79], pulmonary fibrosis [80] and cystic fibrosis [81]. The production of ROS by neutrophils involves this impaired phagocytosis process, in which ROS activate the GTPase ras homolog gene family member A (RHOA) in surrounding phagocytes and reduces apoptotic cell engulfment by neighboring cells [82–85]. Alveolar macrophages from patients with severe asthma and children with poorly controlled asthma are defective in clearing apoptotic cells [86, 87]. As the mainstay of treatment for asthma, corticosteroids not only induce eosinophil apoptosis [88] but also enhance monocyte-derived macrophage engulfment [89]. The mechanism underlying the enhanced clearance seems dependent on the binding of protein S to apoptotic cells and the upregulation of tyrosine-protein kinase MER on the surface of macrophages [90]. Recently, airway epithelial cells have been found to be capable of engulfing neighboring apoptotic cells, and deficiency of this engulfing function increases pro-inflammatory mediator production and exacerbates airway inflammation [91]. Apoptotic cells are well established to induce the synthesis of anti-inflammatory mediators such as TGF-β, prostaglandin E2, and platelet activating factor by macrophages [92, 93].
In summary, apoptotic signaling pathways may be activated by specific PRRs which contrasts with the traditional model. Furthermore, inflammation is affected by neutrophil apoptosis and the clearance of apoptotic cells. Therapeutic induction of neutrophil apoptosis at the inflammatory site may be a powerful pro-resolution intervention and could fulfill the clinical need to prevent the harmful consequences of inflammation.
4 Pyroptosis and Inflammation
Pyroptosis , a form of cell death, is dependent on the activation of caspase-1. Pyroptosis is characterized by the rupture of the plasma-membrane, releasing proinflmmatory intracellular content. Cell lysis during pyroptosis results from caspase-1-mediated processes [94–102]. Plasma membrane pores dependent on caspase-1 dissipate cellular ionic gradients, producing a net increase in osmotic pressure, water influx, cell swelling, and eventual osmotic lysis, followed by release of inflammatory intracellular content [103]. Cell death due to pyroptosis results in a measurable cellular size increase and cleavage of chromosomal DNA [96, 98, 103–106].
The inflammasome, a caspase-1-containing complex that activates the proinflammatory cytokines IL-1β and IL-18 and results in proinflammatory cell death, is one of the drivers of pyroptosis. The inflammasome activates caspase-1 through a Nod-like receptor (NLRP1, 3, 6, 7, 12, NLRC4), AIM2, or Pyrin, all of which contain a CARD or PYD [107, 108]. Many inflammasomes recruit the ASC adaptor via homotypic interactions. Additional ASC molecules are incorporated via CARD-CARD and PYD-PYD interactions, until all ASC molecules are collected into a single focus. The recruitment of procaspase-1 into the ASC focus via CARD-CARD interactions results in its dimerization and proximity-induced autoproteolytic processing into the p10 and p20 subunits. This processed and catalytically active caspase-1 cleaves pro-IL-1β and pro-IL-18.
Studies have shown that ASC specks collect in extracellular space and promote maturation of IL-1β after pyroptosis [109]. In addition, phagocytosis of ASC specks by macrophages induces lysosomal damage and nucleation of soluble ASC as well as activation of IL-1β in recipient cells [109]. These findings indicate that pyroptotic cell-released inflammasomes serve as danger signals promoting enhanced activation of macrophages.
IL-1β and IL-18 are inflammatory cytokines secreted after caspase-1 activation by pyroptotic cells. IL-1β is a potent endogenous pyrogen that stimulates fever, leukocyte tissue migration, and expression of diverse cytokines and chemokines [110]. IL-18 induces IFNγ production and is important for the activation of T-cells, macrophages, and other cell types [111]. Cytokine secretion occurs through caspase-1-dependent pores in the plasma membrane. Pharmacological inhibition of cell lysis does not prevent caspase-1-dependent pore formation and cytokine secretion, suggesting that lysis is not required for the release of active IL-1β and IL-18 [103]. Thus, cytokine secretion and cell lysis are both downstream consequences of caspase-1-dependent pore formation. Notably, caspase-1 activation cannot trigger pyroptosis in all cell types; specifically, epithelial cells use caspase-1 activation to prevent cell death—i.e. caspase-1 activation stimulates lipid production and membrane repair in response to the pore-forming toxins aerolysin and α-toxin [112].
In addition to caspase-1, caspase-11 has also been found to be involved in pyroptosis [113–115]. A recent study revealed that caspase-11 participates in the process of non-canonical inflammasome activation downstream of a cytosolic ligand released from bacteria [116, 117].
Pyroptosis can induce pathological inflammation as a defense against infection. However, exuberant or inappropriate caspase-1 activation and pyroptosis can be detrimental. During infection, caspase-1 activation helps to clear pathogens, such as Salmonella [118, 119], Francisella [120], Legionella [102, 121], Shigella [122], Anaplasma phagocytophilum [123], Burkholderia thailandensis [124], Burkholderia pseudomallei [125] and Listeria [126]. Mutations in nucleotide-binding oligomerization domain (NOD)-like receptor (NLR) proteins can lead to improper caspase-1 activation and can cause hereditary autoinflammatory syndromes [127]. Moreover, caspase-1 is involved in the pathogenesis of several diseases characterized by inflammation and cell death, including myocardial infarction [128], cerebral ischemia [129], neurodegenerative diseases [130], inflammatory bowel disease [131], and endotoxic shock [132].
As one of the most recently recognized types of cell death, pyroptosis exhibits a particular relationship with common pathogens, and clinic inflammatory disease for caspase-1 connects to both cell death and pro-inflammation directly. Pyroptosis and other caspase 1-dependent processes are therefore relevant to our understanding of the pathophysiology of inflammatory disease.
5 Pyronecrosis and Inflammation
Similar to necrosis , pyronecrosis is a cell death process that is dependent on ASC and lysosomal protein cathepsin B but is independent of caspase-1 and -11. HMGB1, a pro-inflammatory mediator, is secreted as a result of pyroptosis [133]. Recent studies have demonstrated that pyronecrosis can be induced by several pathogens, including Neisseria gonorrhoeae [134], Toxoplasma gondii parasitophorous [135], Bacillus anthracis lethal toxin [136] and Staphylococcus aureus [137]. The mechanism underlying pyronecrosis remains unclear at present and requires further investigation.
6 NETosis and Inflammation
A type of polymorphonuclear neutrophil (PMN) death, NETosis releases neutrophil extracellular traps (NETs) [138]. NETs are composed of decondensed chromatin and different neutrophil proteins to form a web-like structure. The purpose of NETs is the capture, neutralization, and clearance of microbes. These large extracellular structures provide a physical barrier to prevent microbial dissemination and increase the local concentration of antimicrobial effectors [139, 140]. NETosis can be categorized by occurrence time, early or late. Late NETosis is more often observed as cell death induced NET release, defined as suicidal NETosis, is a relatively slow process (120–240 min). Suicidal NEToisis is NADPH oxidase-dependent and requires chromatin decondensation, followed by nuclear envelope disintegration and mixing of nucleic acids and granule proteins within a large intracellular vacuole [141]. However, it remains unclear how oxidants participate in the dismantling of the nuclear envelope and mixing of the NET components. Classically, suicidal NETosis occurs following stimulation by phorbol myristate acetate (PMA) through activation of protein kinase C and the Raf–mitogen-activated protein kinase (MEK)–extracellular signal-regulated kinase (ERK) pathway. NADPH assists in the translocation of neutrophil elastase from cytosolic granules into the nucleus, where it aids in chromatin breakdown via histone cleavage. Myeloperoxidase (MPO) is required for chromatin and nuclear envelope breakdown and granular mixing within the NET vacuole. One hundred twenty minutes after intracellular NET formation, the neutrophil outer membrane ruptures, and the mature NET is extruded.
The early form of NETosis occurs rapidly in response to a pathogen, e.g., after in vitro Staphylococcus aureus stimulation for 5–60 min. Early NETosis has also been termed vital NETosis in some studies [142]. In general, NETosis begins when the nucleus loses its characteristic lobulated architecture. Subsequently, nuclear membranes disassemble, and the chromatin decondenses into the cytoplasm while the plasma membrane remains intact. Finally, the plasma membrane bursts, leading to NET released [138]. This process is mainly dependent on ROS, such as superoxides generated by the NADPH oxidase Nox2. This mechanism spares the PMN outer membrane, thereby allowing the PMN to continue to function, even to the point of becoming anuclear. There are three major differences between suicidal NETosis and vital NETosis, including the nature of the inciting stimuli and the timing, the functional capacity of the PMNs during NET release, and the mechanisms employed to make and release NETs. In addition to PMN, NETosis has also been observed in eosinophils and mast cells [143]. Therefore, the more generalized term ‘ETosis’ may be more accurate [144].
Apart from immobilization and capture, NETS are able to directly kill a number of pathogenic bacteria [145–148]. Studies show that bacterial virulence factors can be inactivated by NETs [138]. NETs may also serve to opsonize certain fungi, such as A. fumigatus via long pentraxin 3 [149]. NETs generated from PMNs can inhibit the growth of Aspergillus [145] and kill C. albicanscan, even the opportunistic pathogen P. aeruginosa [150]. The gram-negative bacterium K. pneumoniae is not sufficient to induce NETosis in isolated neutrophils ex vivo but is a good inducer in a mouse lung infection model [151]. Human immunodeficiency virus (HIV)-1 has been shown to induce NETosis through a cell death pathway [152]. Feline leukemia virus (FeLV) was able to inhibit neutrophil activation by inhibiting the activation of PKC to reduce ROS production [153].
NETs and NETosis are associated with many types of inflammation. NETs are observed in both infection- and sterile- acute lung injury (ALI) models related to influenza virus [154, 155], bacteria or bacterial component LPS [156–158], fungi [148, 159, 160], and transfusion [161, 162]. Among them, human neutrophil antigen (HNA)-3a causes the most severe transfusion-related ALI and has been shown to promote NETosis in human neutrophils in vitro [161]. Extracellular neutrophil elastase release via NETosis may be an important cause of lung tissue damage and cystic fibrosis progression [163]. NETs have been shown to form scaffolds in circulation that promote thrombus formation by interacting with the endothelium, platelets, coagulation factors and red blood cells, which cause deep vein thrombosis. IL-8 and ROS release from endothelial cells can recruit and trigger neutrophils to form NETs, which subsequently promote damage to the endothelium through the binding of histones [164].
NETosis is a type of cell death specific to neutrophils that gives neutrophils the capacity to capture numerous viruses and pathogenic bacteria. A deeper understanding of the relationship between NETs and invaders would increase comprehension of inflammation and the processes behind it. Furthermore, NETotic products could be treated as prognostic biomarkers for inflammatory disorders, and whether the products correlate with clinical outcome in a variety of diseases requires further translational investigation.
7 Autophagy and Inflammation
Autophagy is a pathway for the degradation and clearance of subcellular component; this pathway is evolutionarily conserved and genetically regulated [165, 166]. Autophagy has previously been classified as a form of programmed cell death to describe a form of caspase-independent necrosis-like cell death associated with the accumulation of autophagosomes in cells [167]. This classification is now controversial, and the causal relationship between autophagy and cell death remains uncertain [168, 169].
When an autophagic isolation membrane, a phagophore, engulfs a portion of cytoplasm, autophagy formation begins [170]. Beclin 1, the serine/threonine protein kinase ULK1, autophagy-related LC3 proteins, and γ-aminobutyric acid receptor-associated proteins are key regulators of phagophore formation [170]. A phagophore sequesters captured cytoplasmic cargo, and a double-membraned autophagosome is formed following elongation and closure. Autophagosome formation is largely controlled by mammalian target of rapamycin (mTOR). Inhibition of mTOR leads to the interaction between ULK1 and AMPK [171, 172], which in turn recruits the type III PI3 kinase VPS34 to promote the development of autophagosome [173, 174]. The degradation of the captured cargo begins when the double-membraned autophagosome matures into a single membrane-delimited autolysosome [175, 176]. Following this step, lysosomes can be recycled from autolysosomes, thereby permitting the cell to reuse a critical component required for further autophagy.
Autophagy can be activated by PRR signaling induced by DAMPs and PAMPs. For instance, TLRs can cooperate with autophagy in response to PAMPs [177, 178], and NLRs can interact with ATGs to localize autophagy [179, 180]. Inflammatory cytokines such as IL-1 family members [181, 182] and IFNγ [183–185] are also involved in the activation of autophagy, whereas TH2 cell-associated cytokines, IL-4, and IL-13, inhibit autophagy [184].
Multiple studies have confirmed the important role of autophagy during the infection process. Autophagy protects organisms from infectious disease by degrading intracellular bacteria, viruses, and protozoan pathogens [186–188].
Autophagy plays a key role in regulating inflammation; this has been observed in Crohn’s disease, a type of chronic inflammation, and sepsis. Polymorphisms in the genes encoding the autophagy-related proteins Atg2a, Atg4a, Atg4d, death-associated protein, immunity-related GTPase family M protein (IRGM), and ULK-1 have been found to be associated with susceptibility to Crohn’s disease [189–191]. NOD2 mutations cause impairment in autophagosome induction and bacterial clearance [179]. Autophagy formation downstream of NOD2 activation controls IL-1β and IL-6 release [192, 193] and results in the tolerogenic presentation of commensal bacterial components on MHC class II complexes in dendritic cells [180]. Inhibition of autophagy in septic mice boosts inflammatory cytokine levels and increases mortality. This effect may be due to the failure to clear damaged or dysfunctional mitochondria, which activate the NLRP3 inflammasome [194].
Although the relationship between autophagy and cell death remains uncertain, several members of the inflammation process are involved in autophagy. The function of autophagy in related inflammatory diseases requires further investigation. A better understanding of the relevance of the contribution of autophagy to inflammatory diseases has great clinical potential.
8 Trauma Regulation of Cell Death
Several experimental and clinical studies have shown that surgical and trauma injury markedly affects the immune system, including both the specific and the nonspecific immune responses [195–198]. The protective immunity of the hosts may critically depend on an appropriate cytokine balance, proper activation and recruitment of PMNs and monocytes/macrophages, an intact macrophage–T-cell interaction, and an adequate T-helper (Th)1/Th2 conception of T-helper cell activation. The surgical and trauma injury potentially disintegrates these complex regulatory systems and induces the deterioration of immune function [195–198]. Trauma is an important regulator of cell death, not only through damaging tissue and cells, but, more importantly, also through releasing endogenous danger signals that induce regulated cell death, and thereby, influence the development of post-trauma inflammation and subsequent organ dysfunction. Trauma regulation of cell death exhibits complicated mechanisms and multiple facets associated with different post-trauma situations, including temporal and infectious factors.
8.1 Trauma Regulation of Macrophage Autophagy
Patients are especially susceptible to a secondary inflammatory stimulus as a response to multiple organ dysfunction syndrome (MODS ) and systemic inflammatory response syndrome (SIRS) after major trauma and surgery that results in hemorrhagic shock (HS). This increased susceptibility is due to a cell priming mechanism [199]. ALI, a main cause of patient death following HS, is a major component of MODS. Inflammation is self-regulated through the balance of interaction between pro- and counter-inflammatory factors. Alveolar macrophages (AM) are critical to the development of inflammation. Macrophages are activated via families of related PRRs, including TLRs and NLRs [200–203]. NOD2, the product of CARD15, is a member of a growing family of NLRs that have been implicated in the regulation of immune responses and cell death in animals and plants [204, 205]. NOD2 acts as a cytosolic recognition molecule of bacterial peptidoglycan (PGN), which is found on both Gram-positive and Gram-negative bacteria, through specific detection of the conserved muramyl dipeptide (MDP) structure [206]. NOD2 have been shown to associate with RIPK2/RICK, via CARD-CARD interactions, which allow RIPK2 to associate with TRAF6/TAK1 [207]. Subsequent signaling leads to activation of NF-κB and upregulation of inflammatory mediators, such as IL-6 [207]. Studies have also shown that NLRs, including NOD2, regulate autophagic processes during bacterial infection, which are now recognized to influence pro- and anti-inflammatory responses in cells [179, 180].
We demonstrated that HS upregulates NOD2 expression in AM through HMGB1/TLR4 signaling. Upregulated NOD2 subsequently sensitizes AM to respond to NOD2 ligand MDP, which initially leads to augmented inflammation in the lung. NOD2 signaling also induces autophagy in AM, which in turn exhibits a potent anti-inflammatory effect on lung inflammation at later time points, thereby negatively regulating inflammation. However, this anti-inflammatory effect was concealed by HS-activated PMN that migrated into alveoli and counteracted the effects of autophagy in AM (Fig. 2) [208]. This study identifies a previously unrecognized HMGB1/TLR4-NOD2-autophagy axis that serves as a macrophage self-regulatory mechanism governing post-HS inflammatory responses to bacterial products. The findings also explored a novel function of PMN NAD(P)H oxidase-derived oxidant signaling in enhancing HS-primed lung injury. PMN NAD(P)H oxidase activates transcellular oxidant signaling through its ability to counteract the autophagy-induced anti-inflammatory mechanisms, and therefore enhances post-HS lung inflammation and injury. In the broadest sense, these findings may also be valid in other human diseases in which macrophages play a role, including diseases associated with acute and chronic inflammation.
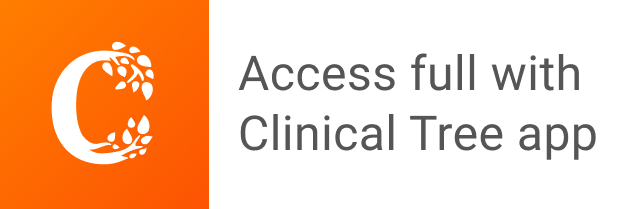