Fig. 16.1
Sequence of events leading to thrombus formation on blood-contacting surfaces (e.g., implanted medical devices)
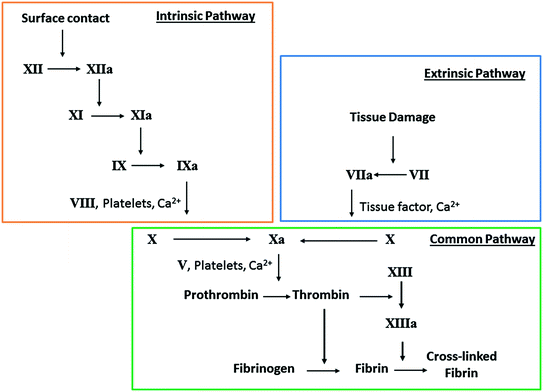
Fig. 16.2
Simplified schematic of the coagulation cascade, where clotting is initiated by either surface contact (intrinsic) or tissue factors (extrinsic) and ultimately converges at the common pathway to form thrombus
Improving Hemocompatibility of Biomedical Devices
In contrast to many polymers used in blood-contacting devices, the endothelial layer lining the human vasculature remains thrombus-free through several control mechanisms: a non-fouling phospholipid coating, membrane bound/released inhibitors of platelet and coagulation factors, as well as an efficient fibrinolytic system that removes fibrin deposits. Many of the major approaches for developing polymeric materials that are more hemocompatible are aimed at decreasing activation of key components of the coagulation cascade. Surface pacification is one approach, where the polymer surfaces aim to minimize protein adsorption (e.g., fibrinogen) and platelet adhesion/activation. Another approach is utilizing active polymer surfaces (e.g., immobilized heparin or nitric oxide (NO) release) that can interact with proteins, cells, and platelets to inhibit parts of the coagulation cascade. Research groups have worked to develop materials with suppressed blood-material interactions (e.g., polymeric materials which exhibit reduce protein and cell adhesion) and materials that mimic the non-thrombogenic endothelium. Polymers for blood-contacting devices are evaluated using various in vitro and in vivo testing methods, where protein and platelet adhesion are common markers to demonstrate the enhanced hemocompatibility properties of the material.
Overview of Current Technology/Strategies
Hydrophilic and Hydrophobic Materials
Protein adsorption on polymer surfaces is a well-recognized initiator of thrombus formation. Protein adsorption is dependent on a variety of material properties including surface charge, surface free energy, surface roughness, and a balance between hydrophobic and hydrophilic groups [10]. Ikada et al. [11] suggested that protein adhesion can be minimized when surface free energy is minimized, which occurs on strongly hydrophilic and strongly hydrophobic surfaces. One widely used approach has been to utilize hydrophilic surfaces in order to reduce protein adsorption and surface thrombogenicity. It has been concluded that hydrophilic polymers exert a steric repulsion effect towards blood proteins and cells [12, 13]. Hydrogels for blood contacting devices have been prepared using poly(vinyl alcohol) (PVA), polyacrylamides (PAAm), poly(N-vinyl pyrrolidone) (PNVP), poly(hydroxyethyl methacrylate) (PHEMA), poly(ethylene oxide) (PEO), poly(ethylene glycol) (PEG), poly(ethylene glycol) monomethyl ether (PEGME), and cellulose [14]. These hydrophilic materials strongly adsorb water, increasing its similarity to biological tissue and producing minimal interface tension with blood [15]. This strongly bound water also prevents cells and proteins from coming into contact with the polymer, reducing their adsorption to the surface [16].
Many hydrogels have poor mechanical properties, thus they have been grafted or coated onto other polymeric substrates and have improved hemocompatibility [15, 17–31]. Immobilization of PEG (–CH2CH2O–) is another popular means to make a polymer more protein and cell resistant [32]. Balakrishnan et al. demonstrated that PEGylated poly(viny chloride) had significantly less fibrinogen and platelet adsorption in comparison to a PVC control [21]. Li et al. reported that poly(poly(ethylene glycol) dimethacrylate) (P(PEGDMA)) grafted on silicone rubber reduced platelet adhesion by 90 % and also reduced plasma protein adsorption [26]. However, platelets still adsorbed on some PEG surfaces during in vivo experiments, despite the low protein adsorption observed during in vitro studies [33]. Plasma oxidation of polyethylene caused increased wettability with increased protein adsorption, but still reduced platelet adhesion [34]. Some hydrogels (e.g., cellulose) typically used as dialysis membranes/fibers are known to be thrombogenic, and therefore it can be concluded that low-protein adhesion does not necessarily result in hemocompatible surfaces [35]. Poly(2-methoxyethyl acrylate) (PMEA), which has been approved by the U.S. Food and Drug administration for medical use, not only exhibits lower protein adsorption and platelet adhesion than other hydrophilic surfaces, but it also reduces the amount of conformational changes that proteins undergo when adsorbed [16, 36]. This is of benefit since denaturation of adsorbed proteins can lead to platelet activation and subsequent thrombus formation, whereas, in contrast, when adsorbed proteins remain in their native confirmation, platelets cannot adhere (see discussion in Sect. “Albumin Coated Surfaces”).
Strongly hydrophobic polymers are also known to have good hemocompatibility properties. Some hydrophobic polymers that are commonly used for medical applications include polyurethanes, silicones, polytetrafluoroethylene (PTFE), poly(vinyl chloride) (PVC), and polyethylene [37]. Very smooth silicone rubber and polyurethanes are known to have good thromboresistant properties [38, 39]. Hydrophobic polymers are conducive to non-specific protein adsorption (e.g., fibrinogen, albumin), and the adsorbed albumin appears to prevent subsequent protein adsorption [40]. It has been shown that increasing surface hydrophobicity also increases the amount of protein adsorbed, which, in turn, decreases the amount of conformational changes, potentially having a role in pacifying the surface [41]. For example, Elast-eon polymers (polyurethane and polydimethylsiloxane copolymers) are reported to have low surface energy and stronger binding to albumin (over fibrinogen) [42]. Alkyl chains have also been grafted on relatively hydrophilic polymers in order to increase the hydrophobicity of the surface [43–45]. Khorasani et al. [46] reported that both superhydrophobic and superhydrophilic surfaces were able to reduce platelet adhesion in comparison to controls. However, materials that are hydrophobic exhibit high protein adsorption and conformational changes of the adsorbed proteins [47, 48]. Polymeric materials with both hydrophilic and hydrophobic domains have also been reported to improve hemocompatibility (see Sect. “Patterned Modifications of Surfaces”). Ultimately, there is still no consensus as to which is better, hydrophilic or hydrophobic surfaces, for blood-contacting biomedical device applications [49].
Albumin Coated Surfaces
It is a widely accepted fact that protein adsorption is the first event that occurs upon foreign surface-blood contact. Human albumin (Alb) is the most abundant protein in the body with a concentration of 35–53 mg/mL in blood plasma. Due to its high concentration and low molecular weight, it is the first protein that adsorbs on the surface of implanted materials. Unlike fibrinogen, albumin is not known to have a peptide sequence that can facilitate binding of the platelet receptors and hence has been used as a coating to block non-specific platelet-surface interactions.
Since the early finding that Alb coated surfaces prevent adhesion of proteins and platelets [50], Alb has been extensively used as one of the strategies to develop hemocompatible surfaces. In one study, Alb was shown to significantly improve short-term thrombogenicity of Dacron arterial prostheses [51]. In another study, Guidoin et al. showed that Alb treatment does not affect the strength of polyester arterial prosthesis, but also found that within 1–2 weeks of implantation the Alb coating begins to disappear [52]. In a comparison of carbon dioxide gas plasma-treated polystyrene (PS-CO2) coated Alb and PS-CO2 treated Alb-heparin conjugate, albumin treated surfaces were found to be more effective in reducing platelet adhesion [53]. Albumin-heparin conjugate surface was also found to be suitable for endothelial cell seeding, which can further improve the hemocompatibility of the surfaces. Mohammad et al. demonstrated that combining Alb with Immunoglobulin G (IgG) results in a significant reduction in platelet adhesion in a 7 day in vitro study [54].
One of the limitations of Alb coating is that other proteins can displace Alb on the surface and reduce the long-term effectiveness of this approach. To prevent this displacement, covalent immobilization of Alb has been reported [55]. Another challenge with the Alb coating approach is that it adsorbs to hydrophobic surfaces more tightly than the hydrophilic surfaces, which necessitates increasing the hydrophobicity of the surface, a modification that is considered undesirable because platelets also adhere strongly to hydrophobic surfaces [56, 57]. Some other limitations of Alb coatings include conformational changes, physical degradation, and challenges with sterilization and shelf stability. In a recent study by the Latour group, it was shown that non-activated platelets can adhere to adsorbed Alb once a critical degree of adsorption-induced unfolding is reached [58, 59]. The platelet response shows a strong correlation with the degree of adsorption induced unfolding, very similar to the platelet adhesion response to adsorbed fibrinogen. These studies demonstrate the potential challenges with Alb coated surfaces, in that, while they may pacify the surface initially, the adsorbed Alb will show a time-dependent conformational change potentially leading to increased platelet adhesion/activation.
Patterned Modifications of Surfaces
Surfaces with patterns at the micro- and nanoscale level have also been investigated for their hemocompatibility properties. Copolymers containing both hydrophilic and hydrophobic domains (ABA-type block copolymers) have exhibited good antithrombotic properties [60–66], where the balance between the hydrophilicity and hydrobphobicity is important to enhance the biocompatibility [67]. Surfaces with hydrophilic/hydrophobic microdomains are reported to create an organized protein structure, albumin adsorbing on hydrophilic domains and fibrinogen adsorbing on hydrophobic domains, which suppresses platelet adhesion/activation [64, 68]. Okano et al. reported that copolymers composed of hydrophilic monomers, 2-hydroxyethyl methacrylate (HEMA) or poly(2-hydroxyethyl methacrylate) (PHEMA), and hydrophobic styrene had excellent thromboresistance properties, in terms of preventing platelet adhesion and deformation, during in vitro experiments [61, 62]. The PHEMA-styrene copolymer was coated on vascular grafts and had an occlusion time of 20 days (vs. 2 days for controls) when implanted in rabbits [61]. However, some of these ABA-type copolymers have the disadvantage that the hydrophilic segments have high surface free energy and bury themselves in the hydrophobic segments [69]. Oyane et al. [60] has reported a new block copolymer (PS-PME3MA) where the hydrophilic blocks remain at the surface when in contact with water. The PS-PME3MA polymer was found to be highly resistant to protein adsorption, cell adhesion, and platelet adhesion/activation.
Another type of surface modification that has been shown to exhibit improved hemocompatibility is attachment of hydrophilic polymer brushes, such as PEO [70, 71] or PEG [72–75]. These hydrophilic polymer brushes create an antifouling surface on the substrate due to steric repulsion. Long-chain polymer brushes have been attached to surfaces using physical adsorption or covalent binding techniques [76]. Polymer brushes that are more densely packed (high graft density) and have longer chain lengths further reduce protein adhesion and platelet adhesion/activation on the surface (Fig. 16.3) [77–79]. Stents coated with a chitosan-PEO coating were tested in an ex vivo porcine model, and had low platelet adhesion (similar to the endothelium) [80]. Dialysis membranes grafted with PEG brushes also had reduced platelet adhesion and improved hemocompatibility [72, 81, 82]. Tetraethylene glycol dimethyl ether grafted on polyethylene tubing was able to significantly reduce plasma protein (both fibrinogen and von Willebrand’s factor) and platelet adsorption, in comparison to control tubing (PVC/Tygon, polyurethane, silicone, and polyethylene) [83]. Rodreguez-Emmenegger et al. recently reported an ultra-low fouling surface using poly[N-(2-hydroxypropyl) methacrylamide] (poly(HPMA)) brushes which was able to maintain the antifouling properties for up to two years of storage [84].
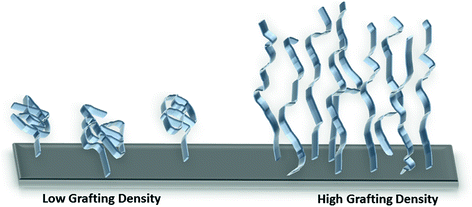
Fig. 16.3
Illustration showing relationship between polymer brush grafting density and chain length
Attached Endothelial Cells
Another approach to making a surface more hemocompatible is to mimic the inner surface of blood vessels by attaching endothelial cells on the artificial surface, which provides an advantage in that the blood will come in contact with a surface that functions just like the endothelium. Endothelial cells (EC) line the inner walls of blood vessels and help in preventing thrombus formation by releasing anti-platelet agents such as nitric oxide (NO) and prostacyclin. Several research groups have used EC seeding on artificial surfaces for various biomedical applications including vascular grafts [85–87], stents [88], resorbable meshes [89], etc.
Williams and co-workers used a pressure sodding method to introduce EC cells into the luminal surface of PTFE grafts before implantation [86]. These grafts were tested for 12 weeks in carotid arteries of dogs. All of the control grafts clotted, whereas 86 % of the EC sodded grafts were found to be patent after the 12 week implantation period. To improve the hemocompatibility of vascular grafts, Taite et al. used NO releasing PU-PEG copolymer containing cell adhesive pentapeptide sequence to promote EC cell adhesion and migration [90]. In another study, Kutryk et al. [88] used xenotransplantation to seed endothelial cells on the surface of the stents to reduce blood contact with the stent surface. Zünd and co-workers, seeded fibroblasts and ECs on polyglycolic acid (PGA) resorbable mesh as a precursor to vessels or cardiac valves [89].
Despite these encouraging results, EC proliferation and seeding on artificial surfaces is complex and still has challenges to overcome. One of the challenges of using the EC seeding approach is the difficulty in growing and maintaining the cells on artificial surfaces [91]. Various studies have been conducted to modify the surfaces in such a way that they can improve and promote endothelial cell adhesion. Kawamoto et al. showed that by plasma treatment of a PU surface, EC adhesion and proliferation can be dramatically improved [92]. In another study, Li and co-workers showed that increased proliferation and cell spreading can be achieved with arg-gly-asp (RGD) peptide grafted surfaces [93]. In yet another study, Yin et al. reported that mussel adhesive polypeptide mimic, containing dihydroxyphenylalanine and L-lysine (MAPDL) with PEG spacer, improved the cell attachment and growth and also reduced platelet adhesion in comparison to the controls [94]. Other challenges associated with the EC seeding approach include complex and expensive experimental procedures and long-term stability issues which still need to be addressed.
Zwitterionic Surfaces
Another effective approach to obtain a hemocompatible surface includes introducing a zwitterionic group, such as phosphobetaine, sulfobetaine or carboxylbetaine, to the substrate material (Fig. 16.4). These zwitterionic materials have been considered as biomimetic, antifouling, and hemocompatible materials because they contain phosphorylcholine-like groups which are present on the lipid bilayers of the cell membranes [95, 96]. Zwitterionic betaines have both anionic and cationic charged moieties in the same side chain which maintains an overall neutral charge. As discussed earlier, PEG and other hydrophilic materials, such as PEO and PHEMA, become hydrated via hydrogen bonding with water to prevent fouling. Zwitterionic materials can bind water molecules even more strongly (induced by electrostatic interactions) and hence can prevent significant change in protein conformation [97, 98].
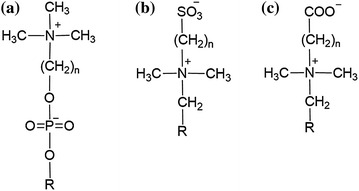
Fig. 16.4
Generic structures of phosphobetaines (a), sulfobetaines (b), and carboxylbetaines (c)
There have been numerous publications showing the potential of zwitterion-based surfaces in resisting protein adsorption [95, 99], platelet adhesion [100–103], and consequently reduced thrombus formation compared to the control materials. Atom transfer radical polymerization (ATRP) is one of the commonly used methods to graft polymer surfaces with zwitterionic groups [97, 104–106]. Recently, various research teams have modified polymers using zwitterionic groups (carboxybetaine and sulfobetaine monomers) to mimic cell membrane surfaces [100, 102, 107, 108]. These surfaces have shown significantly lower fouling and platelet adhesion properties. In an another study, a zwitterionic silane coupling agent, N,N-dimethyl, N-(2-ethyl phosphate ethyl)-aminopropyltrimethyoxysilane (DMPAMS) was synthesized and studied for in vitro hemocompatibility [96]. Surfaces grafted with DMPAMS showed a steep drop in the water contact angle from 48º to 21.5º, indicating a more hydrophilic surface. At 200 mM surface concentration of DMPAMS, activated partial thromboplastin time (APTT) was prolonged from 28.8 s for the control to 37.8 s for the modified surface. Almost no platelet attachment was seen on the DMPAMS modified surface even after 3 h of blood contact. Lee et al. combined PEG with zwitterions for stent coating applications [109]. Significant reduction in protein adsorption and platelet adhesion was observed in addition to a substantial increase (compared to controls) in blood coagulation time for the zwitterionic-PEG grafted stents [109]. Polyurethane catheters grafted with a zwitterionic sulfobetain monomer (N,N-dimethyl-N-methacryloxyethyl-N-(3-sulfopropyl) ammonium, DMMSA) were also able to prevent platelet adhesion when soaked in platelet rich plasma (PRP) for 120 min [110]. Stents coated with phosphorylcholine, such as the commercial Biodiv Ysio ™, have also been shown to exhibit enhanced hemocompatibility and increased endothelialization [111, 112].
Heparin Immobilization
Heparin (Fig. 16.5) is a highly sulfonated, anionic blood polysaccharide that binds to antithrombin III through ionic interactions, which inactivates thrombin and Factor Xa and thereby inhibits blood coagulation. Heparin has been clinically used since 1935 and is one of the most common anticoagulants employed during surgery and treatment of post-operative thrombosis and embolism [113]. However, systemic administration of anticoagulants, such as heparin, increases the risks of hemorrhage, thrombocytopenia, and thrombosis [7]. It is well known that some heparinized and sulfonated materials have anticoagulant activity, resulting in prolongation of the blood clotting time [114]. Heparin immobilized on surfaces also suppresses platelet adhesion and protein adsorption [115]. Heparinization of surfaces is one of the most popular techniques to improve hemocompatibility and has been commercialized and used in the preparation of different medical devices (e.g., catheters, extracorporeal circuits, stents, grafts, etc.) [116, 117].
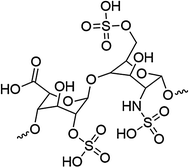
Fig. 16.5
Structure of a disaccharide sequence of heparin
Heparin has been physically adsorbed, as well as ionically and covalently immobilized, on various polymer surfaces [117–122]. Ionic immobilization techniques require cationic residual groups in the polymer which interact with the ionic groups on heparin (e.g., COO−, SO42−, NHSO3 −), while covalent binding of heparin utilizes the hydroxyl, carboxyl, or amino groups of heparin [120]. Heparin that is ionically bound to a surface will slowly be released due to ion-exchange with blood components, and this ultimately shortens the usage lifetime of the material [123]. The success of heparinized materials depends on the covalently bound heparin remaining in its native confirmation and its ability to complex with antithrombin III, which is most efficient when heparin is coupled by endpoint (vs. multipoint) attachment [124]. Michanetzis et al. [120] compared common heparin immobilization techniques, direct [125] and indirect [126] covalent binding, applied to the surfaces of commercially available polymers (silicone rubber, polyethylene, polypropylene, and poly(viny chloride)). While both techniques suffered from a low yield of immobilized heparin in comparison to the initial amount of heparin, the direct method produced a better heparinization yield (10.5 %) and both methods were able to improve the hemocompatibility in terms of reduced platelet activation and, therefore, an increased platelet retention rate. The anticoagulation properties of immobilized heparin have also been improved by using hydrophilic spacers (e.g., PEG), in comparison to heparin immobilized directly to the polymer. This reduces protein adsorption [127, 128]. Polyethylene tubing was modified with immobilized heparin via the method developed by Larm et al. [119] (commercialized as Carmeda ® BioActive Surface) and was able to maintain efficacy for up to 4 months when implanted in pigs [129]. The advantage of heparinized surfaces, in addition to the binding of antithrombin III, is the reduced/selective adhesion of certain plasma proteins, which alters the composition of the surface-adsorbed layer of proteins [130–133]. Heparin-coated devices, however, also suffer challenges, especially for long-term applications, due to heparin’s short half-life and that surface-bound heparin only has ~1 % of the activity of free heparin [134]. Other immobilized direct thrombin inhibitors, such as hirudin, have demonstrated thromboresistant properties as well [116, 135].
Nitric Oxide (NO) Releasing/Generating Polymers
Nitric oxide (NO) is known to be a potent inhibitor of platelet activation and adhesion. Healthy endothelial cells exhibit an NO flux of 0.5−4.0 × 10−10 mol cm−2 min−1 [136]. Polymeric materials with an NO flux that is equivalent to this level are expected to have similar anti-thrombotic properties. Nitric oxide is highly reactive under physiological conditions, thus a wide range of NO donor molecules, with functional groups that can store and release NO, have been studied for potential biomedical applications. Various reviews have been published that are devoted to a comprehensive discussion of different NO releasing/generating materials and their many potential biomedical applications [137–144]. The two major approaches to achieving localized NO release from polymeric surfaces are to (1) incorporate NO donor molecules or functional groups (e.g. diazeniumdiolates or S-nitrosothiols) into the polymer that will release the bound NO under physiological conditions (NO releasing polymers (NOrel)), or (2) incorporate catalysts into the polymers which can react with endogenous S-nitrosothiol (RSNO) species (present in blood) to locally generate NO (NO generating polymers (NOgen)) (Fig. 16.6). Nitric oxide has many other biological roles, including its antimicrobial action, which could potentially be utilized to also reduce the infection and biofilm formation that plagues many implantable biomedical devices. Thus, NO releasing/generating chemistries have the potential to create a dual-functioning material that is both antithrombotic and antimicrobial. Duration of the NO release can be tailored to fit the specific biomedical application of the device, and NO-generating materials can continuously generate NO in the presence of endogenous RSNO species (e.g., S-nitrosoglutathione (GSNO), S-nitrosocysteine (CysNO), etc.).
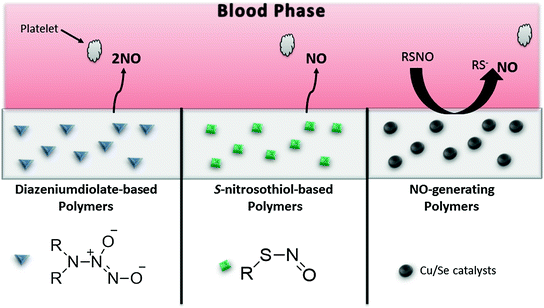
Fig. 16.6
Types of NO-releasing/generating polymers, where the NO that is released/generated can prevent activation of platelets that approach the polymer surface. Diazeniumdiolate-based materials undergo proton and thermal driven mechanisms to release NO. S-nitrosothiol (RSNO)-based polymers can release NO in the presence of heat, light, or metal ions (e.g., Cu+). NO-generating materials consist of immobilized catalysts (e.g., Cu or Se compounds) that generate NO from endogenous RSNOs
A wide variety of NO donor molecules have been investigated. The two most widely studied and used in combination with biomaterials are diazeniumdiolates and S-nitrosothiols. Diazeniumdiolates, also called NONOates, are relatively stable species that undergo a proton or thermally driven mechanism to release two molecules of NO per diazeniumdiolate molecule. Diazeniumdiolates have been a popular NO donor that can easily be dispersed within polymers to facilitate localized NO release [145–149]. Mowery et al. [145] reported that water-soluble diamine-based diazeniumdiolates (e.g., DMHD/N2O2, diazeniumdiolated linear polyethylenimine (LPEI/N2O2)) leached from polymer matrices. Significant leaching of the NO donor species can result in non-localized NO release where the therapeutic action of NO occurs downstream from the biomedical device. Another concern with diazeniumdiolated-based polymers is the formation and leaching of some potentially carcinogenic decomposition products (e.g., N-nitrosamines) that are not intended for release into the bloodstream [145, 150]. To overcome the leaching concerns, strategies to covalently bind diazeniumdiolated functional groups to polydimethylsiloxane (PDMS) [151], xerogels [152–154], medical-grade polyurethanes [137, 155, 156], silica nanoparticles [157, 158], dendrimers [159–161], and other nanomaterials [138] have also been reported. Zhang et al. [151] covalently linked diaminoalkyltrimethoxysilane (DACA) to polydimethylsiloxane and then loaded this materials with NO under high pressure to form the diazeniumdiolated coating, DACA/N2O2-SR, which released NO for up to 20 days. The DACA/N2O2–SR was coated on extracorporeal circuits (ECC) and was able to reduce platelet consumption and thrombus formation during the 4 h blood flow in a rabbit model.
Attempts have also been made to add polymer top coats and/or create more lipophilic diazeniumdiolated species to minimize leaching into the aqueous phase and therefore maintain the localized NO release at the blood-polymer interface [147, 150, 162, 163]. Poly(vinyl chloride) and polyurethane have been doped with the lipophilic diazeniumdiolated dibutylhexanediamine (DBHD/N2O2) [147, 148, 164, 165]. Vascular grafts coated with DBHD/N2O2–doped polyurethane had significantly less thrombus formation than controls after 21 days implantation in sheep [148]. However, the loss of NO from DBHD/N2O2 creates free lipophilic amine species within the polymer that react with water, thereby increasing the pH within the polymer phase and effectively turning off the NO release. A recent report demonstrated that NO release can be prolonged, by using poly(lactic-co-glycolic acid) (PLGA) additives, for up to 14 days from poly(vinyl chloride) (PVC) doped with diazeniumdiolated dibutylhexanediamine (DBHD/N2O2) (Fig. 16.7) [165]. The ester linkages of the PLGA hydrolyze in the presence of water, producing lactic and glycolic acids that can act as proton sources to control the NO release from DBHD/N2O2-doped polymers. PLGAs can have varying hydrolysis rates, which is primarily determined by the copolymer ratio, the end group chemistry (either a free carboxylic acid or ester end group), and molecular weight. Handa et al. also demonstrated the importance of the inherent hemocompatibility of the base polymer (in which the NO chemistry is incorporated) [166]. By incorporating the same DBHD/N2O2 and PLGA chemistry into the Elast-eon E2As polymer (a copolymer with a mixed soft segment of poly(dimethylsiloxane) and poly(hexamethylene oxide) with a methylene diphenyl isocyante (MDI) hard segment), which inherently is more hemocompatible than PVC, the platelet count after 4 h of ECC (97 ± 10 % of baseline) was significantly improved over the PVC/DOS-based coating (79 ± 11 % of baseline) [165, 166].
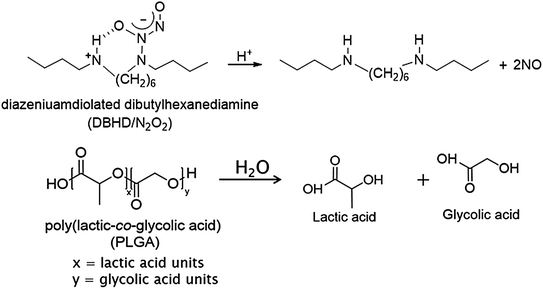
Fig. 16.7
Key reactions involved in NO release from diazeniumdiolated dibutylhexanediamine (DBHD/N2O2) in the presence of poly(lactic-co-glycolic acid) additive, that provides additional protons to drive the NO release reaction
Another widely studied class of NO donor molecules is S-nitrosothiol (RSNO) species (examples shown in Fig. 16.8). Physiological RSNOs, including S-nitrosoalbumin, S-nitrosocysteine (CysNO), and S-nitrosoglutathione (GSNO), are considered an endogenous reservoir of NO in vivo [167–169]. Other synthetic RSNOs, such as S-nitroso-N-acetylpenicillamine (SNAP) and S-nitroso-N-acetylcysteine (SNAC), have been shown to exhibit significant antimicrobial and antithrombotic effects [170–173]. It has also been demonstrated that RSNOs are both vasodilators and potent inhibitors of platelet aggregation [174–176]. RSNOs undergo thermal decomposition to release NO and produce a corresponding disulfide species (RSSR). The NO release from RSNOs can also be catalyzed by metal ions (e.g., Cu+) [177] and by light, through irradiation at energies that correspond to the S-nitroso absorption bands at 340 and/or 590 nm [178–180]. Incorporation of RSNOs into polymers can extend the utility of these NO donors to be applicable in biomedical devices.
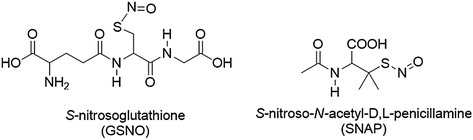
Fig. 16.8
Structures of example S-nitrosothiols species, S-nitrosoglutathione (GSNO) and S-nitroso-N-acetyl-D,L-penicillamine (SNAP)
Low molecular weight RSNOs have been non-covalently dispersed within various polymer matrices [181–187]. Seabra et al. [182] prepared GSNO-doped poly(vinyl alcohol) and poly(vinyl pyrrolidone) blended films. This polymer matrix provided a stabilization effect on GSNO decomposition, in comparison to aqueous solutions of GSNO. However, due to leaching, 90 % of the NO was released during the first 24 h under physiological conditions. SNAP-doped polyurethanes (Tecoflex SG-80A and Tecophillic SP-60D-60) also exhibit rapid leaching of SNAP when soaked in buffer [186]. The rapid leaching of the RSNOs significantly shortened the duration of the NO release from these materials. Therefore, strategies to synthetically bind RSNO functionalities to the polymer backbone have also been explored. S-Nitrosothiol functionalities have been covalently bound to polymers such as xerogels [188, 189], polyurethanes [137, 190, 191], degradable polyesters [192–196], polyester/poly(methyl methacrylate) blends [197], poly(vinyl methyl ether-co-malic anhydride) (PVMMA) and poly(vinyl pyrrolidone) (PVP) [198], and poly(dimethylsiloxane) [199]. Dendrimers [200], fumed silica particles [180, 191], and silica nanoparticles [155, 156, 201] have also been synthetically modified with covalently bound RSNO functionalities and these materials can be doped into various polymer matrices to create coatings for biomedical devices.
Many of the RSNO-modified materials reported to date have not proven clinically useful due to their limited NO release lifetimes, low conversion to RSNO during synthesis, or lack of RSNO stability during storage. Recently, the RSNO-doping method has been revisited, utilizing more hydrophobic polymers. GSNO-doped Tygon, a proprietary plasticized poly(vinyl chloride) polymer, exhibited minimal leaching of GSNO during soaking under physiological conditions [187]. Incorporating SNAP into Elast-eon E2As created an NO-releasing polymer that is stable during storage (even at elevated temperatures), locally delivers NO under physiological conditions, significantly reduces the leaching of SNAP, and preserves platelets during a 4 h ECC study [186].
Due to the limited NO reservoir from NO donors that can be incorporated into polymers (either covalently bound or non-covalently dispersed), these NO releasing materials typically have a finite duration of NO release. Another approach to achieving localized NO delivery at a polymer/blood interface for a longer period of time is to use NO generating (NOgen) materials that utilize endogenous RSNOs and/or nitrite to locally generate NO at the blood-polymer interface. Various thiol-containing species (L-cysteine, 2-iminothiolane, and cysteine polypeptide) have been immobilized on polyethylene terephthalate and polyurethane, where the free thiol undergoes a transnitrosation reaction with circulating RSNOs (e.g., S-nitroso-albumin) [202, 203]. The resulting CysNO on the PET and PU surface decomposes to elevate the NO levels locally at the polymer/blood interface, which reduces platelet adhesion on the surface by more than 50 %. Other NOgen materials consist of catalysts (e.g., Cu (I/II) complexes or organoselenium species) that are immobilized within the polymer and are capable of locally generating NO from endogenous RSNOs and/or nitrite. Copper (II) sites within a polymer can be reduced to Cu(I) by endogenous reducing agents that are present in the blood (e.g., thiols, ascorbate). The Cu(I) sites then can reduce endogenous RSNOs in the blood (e.g., GSNO, CysNO, etc.) to NO and free thiolate anions in a catalytic manner [177]. Lipophilic copper complexes that were incorporated into polymers were able to reduce physiological levels of RSNOs and nitrite to locally generate NO [204–207]. Another reported NOgen polymer consists of copper nanoparticles (Cu0) dispersed within a hydrophilic polymer (Tecophillic SP-60D-60), which was evaluated using a rabbit model for extracorporeal circulation (ECC) [208]. However, continuous infusion of SNAP was needed to supplement the endogenous RSNO levels in order to achieve good efficacy in reducing thrombus formation.
Organoselenium species have been studied as mimics of glutathione peroxidase (GPx), a selenoenzyme that protects cells from oxidative stress by reducing hydroperoxides using glutathione (GSH) [209], and investigated for their ability to generate NO from RSNOs [210]. Selenium catalysts are highly selective for reduction of RSNOs and exhibit no catalytic activity for nitrite or nitrate reduction [211]. Low molecular weight organoselenum species, selenocystamine (SeCA) and 3,3′-diselenidedipropionic acid (SeDPA), were immobilized on cellulose filter paper and polyethylenimine (PEI), demonstrating potential dialysis membrane applications [212]. In the presence of reducing agents at physiological pH, the diselenides can be converted into selenolates, which are the key intermediates that can reduce RSNOs into NO and thiolates [212]. Selenium species were also incorporated into layer-by-layer coatings containing alternating layers of sodium alginate (Alg) and selenium-modified PEI. Alg-PEI layer-by-layer films modified with SeDPA were able to generate physiological levels of NO from GSNO and also exhibited minimal leaching of catalytic sites after soaking in blood [211]. The Alg-PEI layer-by-layer coating was also modified to immobilize ebselen (2-phenyl-1,2-benzisoselenazol-3-(2H)-one), an aromatic selenium species with good GPx activity, and coated on catheters [213]. These selenium-based materials were able to generate physiological levels of NO from RSNOs; however, some reduction of NO flux was observed after soaking in blood, potentially from catalytic sites being blocked by adsorbed plasma proteins or low levels of catalyst species leaching into the solution. These NOgen polymers have an advantage in that they could potentially generate NO at the blood-polymer interface indefinitely, provided that the blood in contact with the polymer has adequate levels of RSNOs present at all times [214].
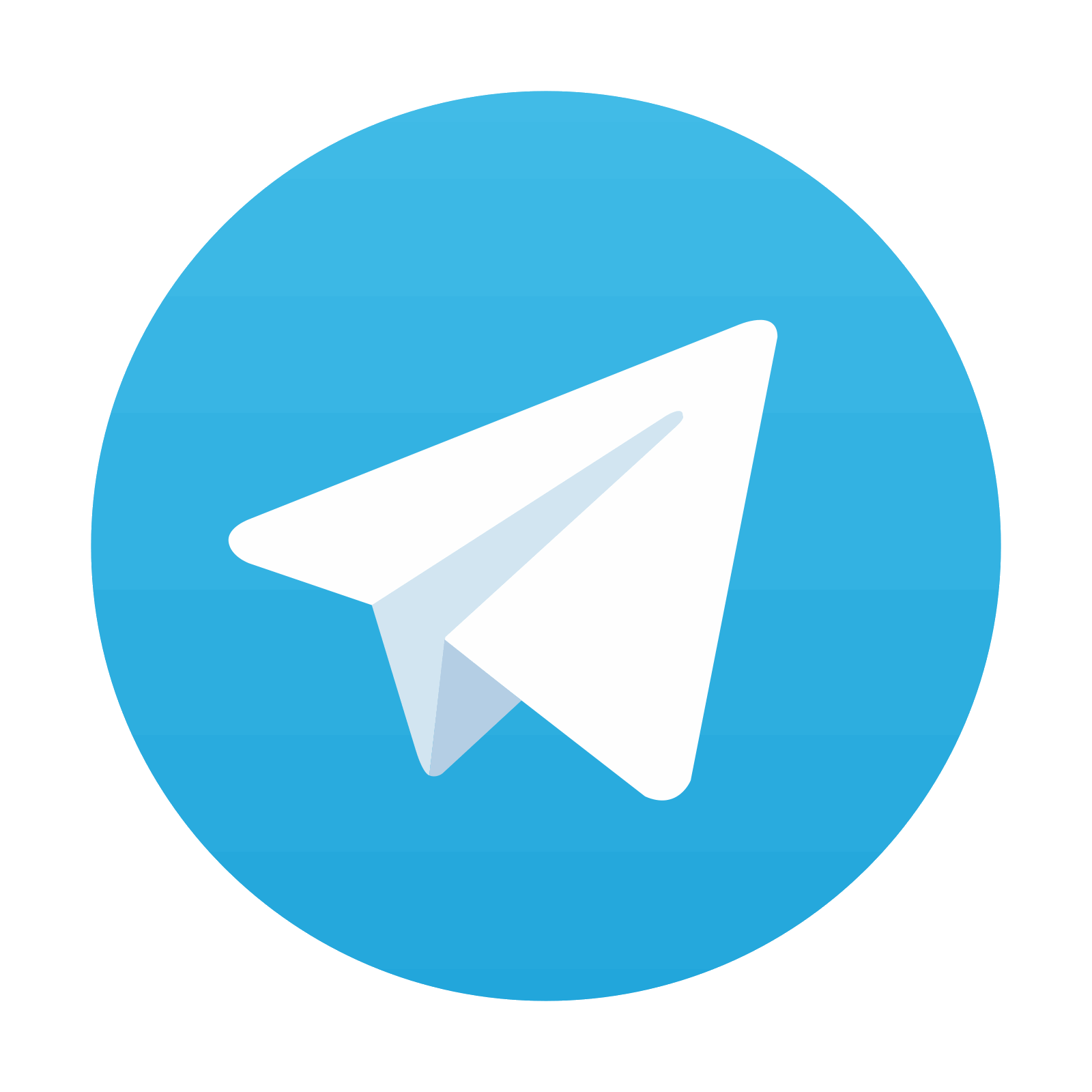
Stay updated, free articles. Join our Telegram channel
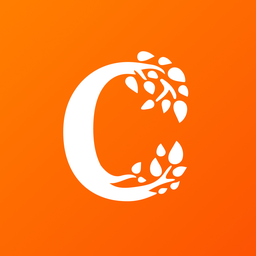
Full access? Get Clinical Tree
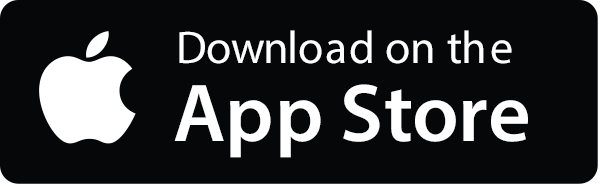
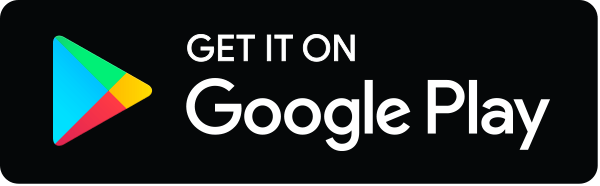