1.2.7 Radical-Based Palladium-Catalyzed Bond Constructions
Y. Li, W. Xie, and X. Jiang
General Introduction
During the evolution of organic chemistry, palladium catalysts have played an important and irreplaceable role in studies on carbon–carbon[1–7] and carbon–heteroatom[8–10] bond formation. Beyond the methodological studies, palladium-catalyzed reactions have also been widely applied in the preparation of natural products,[11] pharmaceuticals, agrochemicals, and materials, even on large scale.[12] Palladium(0) and palladium(II) species are frequently used as the catalysts and considered as active intermediates, participating in oxidative addition and reductive elimination steps in two-electron-transfer processes.[1–15] Throughout the development of palladium chemistry, an increasing number of single-electron-transfer procedures have been proposed and carefully studied, which mainly involve palladium(I)[16] and palladium(III)[17] species. The focus of this chapter is on radical-based palladium-catalyzed bond constructions in organic synthesis.
1.2.7.1 Method 1: Reactions Involving Palladium(I) Species
1.2.7.1.1 Variation 1: Synthesis of Organometallic Palladium(I) Complexes
Various palladium(I) complexes have been successfully synthesized, most of which exist as dimers. In previously reported reactions starting from palladium(I) complexes, the palladium species tended to undergo a single-electron oxidation to generate the corresponding palladium(II) complexes in monomeric or dimeric form. Besides the common ligandexchange reactions, palladium(I) complexes have been transformed with hydrogen, carbon monoxide,[18,19] oxygen,[20] and even ammonia gas,[21] which has helped to further the understanding of palladium chemistry.
The complexes 1 ({PdX[P(t-Bu)3]}2; X = Br, I) are palladium(I) dimers of great significance that have been successfully isolated and transformed (▶ Scheme 1).[18,19] When the dimer 1 (X = Br) is stirred under a hydrogen or carbon monoxide atmosphere, the new palladium hydride species 2 and CO-bridged palladium complex 3, respectively, can be isolated and characterized. In addition, dimer 1 (X = Br) also reacts with terminal alkynes to produce polyethylene derivatives. When dimer 1 (X = I) is reacted with 1,2-disubstituted alkynes (diethyl or dimethyl but-2-ynedioate), a new trinuclear palladium species is formed.[19] Furthermore, an isonitrile also reacts efficiently with dimers 1 to generate new palladium(I) dimers 4, which have four isonitrile units coordinated.
X | Yield (%) | Ref |
Br | 74 | [19] |
I | 58 | [19] |
The palladium(I) dimer 1 (X = Br) can be reacted with aerial oxygen to produce the Pd–O–Pd bridge complex 5 through dual intramolecular C—H activation (▶ Scheme 2).[20] It has been proposed, but not yet clearly confirmed, that the mechanism might include three steps: (1) coordination between the palladium(I) dimer and molecular oxygen with cleavage of the O=O bond; (2) intramolecular activation of two C—H bonds; and (3) the formation of new C—O bonds. In addition, it is not yet clear whether the two oxygen atoms originate from the same molecule of oxygen.
The photochemical homolysis of Pd—C bonds has been observed with the PNP-ligated palladium–alkyl complexes 6, which form the (PNP)Pd—Pd(PNP) dimers 7 with a single Pd—Pd bond (▶ Scheme 3).[21] X-ray diffraction reveals that each palladium center is four-coordinate with a distorted square-planar environment. In addition, electron paramagnetic resonance (EPR) experiments have helped to reveal that the PNP–palladium monomer is reversibly produced in solvent. In fact, thermolysis or photolysis of a 1:1 mixture of 7 (R1 = F)and 7 (R1 = Me) in benzene-d6 results in the formation of a ca. 1:1:2 mixture of complexes 7 (R1 = F), 7 (R1 = Me), and 8. More importantly, the palladium(I) dimers 7 react efficiently with dihydrogen, water, or ammonia via binuclear oxidative addition (▶ Scheme 4).[21] The reaction with ammonia represents a new mode of activation.
When the palladium(I) dimer 7 (R1 = F) reacts with oxygen (1 atm, >10 equivalents) under irradiation by sunlight, it affords the palladium superoxide complex 9 in 95% yield within 1 minute (▶ Scheme 5).[22] The mixture undergoes a rapid color change from the green of complex 7 (R1 = F) to the orange of complex 9. When the oxygen amount is less than 10 equivalents, the formation of complex 10 can be observed. By combining complex 7 (R1 = F) and 9 in a ca. 1:2 ratio, it is possible to generate the complex 10 in relatively pure form. When the solution of complex 9 was concentrated, and the residue was redissolved and then irradiated, complex 10 was again detected, which further confirms the equilibrium between complexes 9 and 10. In summary, the reaction between oxygen and dimer 7 (R1 = F) is irreversible, but an equilibrium exists between oxygen, complex 9, and complex 10 (▶ Scheme 5).

When the solvated complexes [Pd2(NCMe)6]X2 11 [X = BF4, NH2{B(C6F5)3}2] react with 2-substituted 1,8-naphthyridines, various di- or trinuclear palladium(II) cyclometalated complexes are obtained through C—H/Br activation under mild conditions (▶ Scheme 6).[23]

1.2.7.1.2 Variation 2: Reactions Involving Palladium(I) Precatalysts
Most commonly, palladium complexes have been introduced into reaction systems as palladium(0) or palladium(II) species. Since the investigation of palladium(I) catalysts, it has been shown that palladium(I) generally exhibits more efficiency in C—C and C—N bond-forming transformations, which is attributed to the easy generation of active palladium(0) species from the palladium(I) dimer complexes.[24–27] Suzuki couplings, Buchwald–Hartwig aminations, carbonylation couplings, and α-arylation of carbonyl compounds can all be achieved using palladium(I) precatalysts under mild conditions (room temperature) in shortened time (minutes). However, the development of other common cross coupling, oxidative coupling, reductive coupling, and C—H activation reactions is still to be achieved.
The seminal report of a reaction involving a palladium(I) precatalyst is that from Hartwig’s group in 2002.[24] Air-stable palladium(I) dimers have been utilized to catalyze the coupling between various aryl bromides/chlorides and amines to give arylamines 12 (▶ Scheme 7). The reactions are complete within minutes at room temperature, with excellent yields. The aryl halides can have an electron-withdrawing or electron-donating substituent; ortho-substitution is also tolerated. The amines can be secondary aliphatic ones, or primary or secondary aryl ones. Furthermore, the coupling between aryl bromides and phenylboronic acid has been presented, resulting in biaryls 13 (▶ Scheme 8).
Ar1 | X | R1 | R2 | Catalyst | Yield (%) | Ref |
4-Tol | Cl | (CH2)2O(CH2)2 | {PdBr[P(t-Bu)2(1-adamantyl)]}2 | 92 | [24] | |
4-NCC6H4 | Cl | Bu | Bu | {PdBr[P(t-Bu)2(1-adamantyl)]}2 | 93 | [24] |
4-O2NC6H4 | Cl | Bu | Bu | {PdBr[P(t-Bu)3]}2 | 97 | [24] |
4-t-BuO2CC6H4 | Cl | Bu | Bu | {PdBr[P(t-Bu)3]}2 | >99 | [24] |
4-t-BuC6H4 | Br | Bu | Bu | {PdBr[P(t-Bu)3]}2 | 96 | [24] |
4-t-BuC6H4 | Br | Me | Ph | {PdBr[P(t-Bu)2(1-adamantyl)]}2 | 98 | [24] |
4-t-BuC6H4 | Br | Ph | Ph | {PdBr[P(t-Bu)3]}2 | 96 | [24] |
Ar1 | Yield (%) | Ref |
4-Tol | 95 | [24] |
2-NCC6H4 | 92 | [24] |
2-F3CC6H4 | 90 | [24] |
2-MeOC6H4 | 96 | [24] |
In 2004, new palladium(I)–palladium(I) dinuclear complexes 14 with one bridging halide were synthesized, in which only one phosphine is retained in the dinuclear core.[25] An unprecedented μ2-η3:η3 coordination mode between a phenyl ring of the biphenyl-2-yldi-tert-butylphosphine ligand and the palladium(I) unit was present in the complexes (▶ Scheme 9). Furthermore, the catalytic ability in the amination of aryl halides was concisely investigated (▶ Scheme 10); both complexes efficiently catalyze the coupling between an aryl bromide/chloride and primary or secondary arylamines to give diarylamines 15.
Scheme 10 Application of a Palladium(I)–Palladium(I) Dinuclear Complex in Cross Coupling between Aryl Halides and Amines[25]

Ar1 | X | R1 | R2 | Catalyst | Time (h) | Yield (%) | Ref |
4-t-BuC6H4 | Br | Ph | Ph | 14 (Z = Br) | 19 | 86 | [25] |
4-t-BuC6H4 | Br | Ph | Ph | 14 (Z = Cl) | 19 | 76 | [25] |
4-Tol | Cl | 4-Tol | H | 14 (Z = Br) | 3 | 78 | [25] |
4-Tol | Cl | 4-Tol | H | 14 (Z = Cl) | 3 | 81 | [25] |
The palladium(I)-catalyzed amination of aryl bromides has been investigated with a focus on the use of secondary alkyl(aryl)amines and aryl bromides bearing electron-donating and electron-withdrawing groups (▶ Scheme 11).[26] The reactions using the palladium(I) dimer were conducted in parallel with the use of palladium(II) acetate/tri-tert-butylphosphine; generally, the reactions with the palladium(I) dimer as catalyst afford better yields of amines 16.
Ar1 | R1 | Yielda (%) | Ref |
Ph | Cy | 93 (86) | [26] |
3-MeOC6H4 | Cy | 94 (89) | [26] |
4-FC6H4 | Cy | 77 (71) | [26] |
2-Tol | Cy | 60 (52) | [26] |
Ph | t-Bu | 92 (87) | [26] |
3-MeOC6H4 | t-Bu | 91 (90) | [26] |
4-FC6H4 | t-Bu | 87 (87) | [26] |
2-Tol | t-Bu | 61 (12) | [26] |
a Yields using Pd(OAc2) (1 mol%) and t-Bu3P (1 mol%) are given in parentheses. |
Control of the chemoselectivity of a palladium(I)-catalyzed Suzuki coupling has been realized by adjusting solvent polarity (▶ Scheme 12).[27] Thus, reaction of 4-chlorophenyl trifluoromethanesulfonate in a polar solvent (acetonitrile) produces the C—OTf insertion product 17 (X = Cl) selectively; in contrast, use of the less-polar solvent tetrahydrofuran provides the C—Cl insertion product 17 (X = OTf). In addition, regioselective coupling has also been achieved with hetaryl halides 18 (▶ Table 1). In mechanistic studies,31P NMR analysis and DFT calculations have been used to show that a Pd(I)L monomer is the real catalyst, and not the dimer. Detailed computational studies suggest that the active catalyst is generated through a reduction instead of homocleavage or direct disproportionation of the precatalyst. Meanwhile,31P NMR spectroscopy has confirmed that the combination of an arylboronic acid, potassium fluoride, and water triggers the generation of bis(tri-tert-butylphosphine)palladium(0) (▶ Scheme 13). At the same time, a black precipitate forms, which is most likely palladium black. This phenomenon is assigned to the deactivation of catalyst and the incomplete conversion of aryl chlorides in palladium(I)-dimercatalyzed Suzuki couplings.
Scheme 12 Suzuki Coupling of 4-Chlorophenyl Trifluoromethanesulfonate Using a Palladium(I) Catalyst in Different Solvents[27]

Solvent | X | Yield (%) | Ref |
THF | OTf | 76 | [27] |
MeCN | Cl | 78 | [27] |
Starting Materials | Product | Yield (%) | Ref | |
Hetarene | Boronic Acid | |||
![]() | ![]() | ![]() | 90 | [27] |
![]() | ![]() | ![]() | 92 | [27] |
![]() | ![]() | ![]() | 82 | [27] |
![]() | ![]() | ![]() | 91 | [27] |
![]() | ![]() | ![]() | 87 | [27] |
![]() | ![]() | ![]() | 94 | [27] |
Scheme 13 Reactivity of 4-Chlorophenyl Trifluoromethanesulfonate with Bis(tri-tert-butylphosphine)palladium(0)[27]

The palladium(I)-catalyzed carbonylative coupling of aryl halides and amines has been achieved under an atmospheric pressure of carbon monoxide (▶ Scheme 14).[28] The reactions yield the desired products 20 in moderate to good yields within 10 minutes. Aryl iodides are better substrates than aryl bromides, and can be transformed with a lower catalyst loading and at a lower temperature. In light of the high efficiency, this system has been successfully applied in synthesizing radiolabeled amides 21 using11CO gas (▶ Scheme 15).
Ar1 | X | R1 | R2 | Solvent | Catalyst (%) | Temp (°C) | Yield (%) | Ref |
Ph | Br | H | Bn | mesitylene | 10 | 150 | 46 | [28] |
4-MeOC6H4 | Br | H | Bn | mesitylene | 10 | 150 | 46 | [28] |
2-MeOC6H4 | Br | H | Bn | mesitylene | 10 | 150 | 46 | [28] |
![]() | I | (CH2)5 | toluene | 2.2 | 100 | 82 | [28] |
Ar1 | X | R1 | R2 | Catalyst | Trapped Radioactivity (%) | 11C Amide RCPa (%) | 11C Amide RCYb (%) | Ref |
Ph | Br | H | Bn | {PdI[P(t-Bu)3]}2 | 87 | 81 | 70 | [28] |
Ph | Br | H | Bn | {PdI[P(t-Bu)3]}2 | 72 | 68 | 47 | [28] |
![]() | I | (CH2)5 | {PdI[P(t-Bu)3]}2 | 78 | 88 | 69 | [28] | |
a RCP = radiochemical purity. | ||||||||
b RCY = radiochemical yield. |
The cross coupling between aryl bromides and esters can also be catalyzed by {PdBr[P(t-Bu)3]}2 in a process promoted by lithium dicyclohexylamide (▶ Scheme 16).[29] The catalyst loading is 0.05–0.5 mol%. The aryl bromides can be substituted by electron-withdrawing or electron-donating groups. In addition, pyridyl and thienyl bromides are also compatible. However, the choice of esters is limited to tert-butyl propanoate, methyl 2-methylpropanoate, and tert-butyl acetate. It is worth noting that reactions with all three esters can be conducted on a 10-gram scale.
R1 | R2 | R3 | Ar1 | {PdBr[P(t-Bu)3]}2 (mol%) | Yield (%) | Ref |
H | H | t-Bu | 4-t-BuC6H4 | 0.20 | 83 | [29] |
H | H | t-Bu | 4-F3CC6H4 | 0.40 | 73 | [29] |
H | H | t-Bu | 4-FC6H4 | 0.40 | 82 | [29] |
Me | H | t-Bu | 4-t-BuC6H4 | 0.04 | 87a | [29] |
Me | H | t-Bu | 4-FC6H4 | 0.20 | 88 | [29] |
Me | H | t-Bu | 2,4,6-Me3C6H2 | 0.05 | 72 | [29] |
Me | Me | Me | 4-t-BuC6H4 | 0.05 | 72 | [29] |
Me | Me | Me | 4-F3CC6H4 | 0.50 | 60 | [29] |
Me | Me | Me | 2-pyridyl | 0.50 | 71 | [29] |
Me | Me | Me | 3-thienyl | 0.50 | 75 | [29] |
a Reaction was performed on a 40-mmol scale. |
Arylamines 12; General Procedure:[24]
In a drybox, the Pd catalyst (0.005 M in THF), t-BuONa (144.0 mg, 1.50 mmol), an aryl halide (1.00 mmol), and an amine [1.05 mmol in THF (1 mL)] were added to a vial containing a stirrer bar. The vial was sealed with a cap containing a PTFE septum and removed from the drybox. The mixture was then stirred at rt for 15 min. After this time, H2O (1 mL) was added into the vial, and the mixture was extracted with CH2Cl2. The organic layer was dried (MgSO4) and concentrated, and the residue was purified by column chromatography.
Biaryls 13; General Procedure:[24]
In a drybox, {PdBr[P(t-Bu)3]}2 (0.005 M in THF), KOH (168.0 mg, 3.0 mmol), PhB(OH)2(1.08 mmol), and an aryl bromide [1.00 mmol in THF (1.5 mL)] were added to a vial containing a stirrer bar. The vial was sealed with a cap containing a PTFE septum and removed from the drybox. The mixture was then stirred at rt for 15 min. After this time, H2O (1 mL) was added into the vial, and the mixture was extracted with CH2Cl2. The organic layer was dried (MgSO4) and concentrated, and the residue was purified by column chromatography.
Arylamines 15; General Procedure:[25]
To an argon-filled Schlenk tube containing a mixture of the catalyst 14 (0.01 mmol), t-BuONa (1.4 mmol), an aryl halide (1.0 mmol), and an amine (1.2 mmol) was added THF (1 mL). The resulting mixture was stirred at rt until the aryl halide was consumed, and then it was extracted with CH2Cl2. The organic layer was dried (MgSO4) and concentrated, and the residue was purified by column chromatography (silica gel, hexane/EtOAc).
Alkyldiarylamines 16; General Procedure:[26]
To an argon-flushed, three-necked flask, containing Pd(OAc)2 (12.8 mg, 0.057 mmol) or {PdBr[P(t-Bu)3]}2 (22.0 mg, 0.0285 mmol), t-BuONa (1.64 g, 17.1 mmol), an aniline (5.7 mmol), and an aryl bromide (6.9 mmol), was added dry, degassed toluene (20 mL). After stirring at rt for 15 min, t-Bu3P [only in the cases where Pd(OAc)2 was used; 11.5 mg, 0.057 mmol] dissolved in toluene was added. Then, the mixture was heated to 108–110 °C and kept at the same temperature for 1 h. After cooling to rt, the reaction was quenched with H2O (10 mL), and then the organic layer was washed with H2O and concentrated. The crude product was purified by chromatography (silica gel).
2′-Methyl-[1,1′-biphenyl]-4-yl Trifluoromethanesulfonate (17, X = OTf) or 4′-Chloro-2-methyl-1,1′-biphenyl (17, X = Cl); General Procedure:[27]
A N2-filled dry reaction vessel was charged with 4-chlorophenyl trifluoromethanesulfonate (276.0 mg, 1.1 mmol), 2-tolylboronic acid (146 mg, 1.1 mmol), and KF (186 mg, 3.2 mmol). After the vessel was transferred to a drybox, THF or MeCN (2 mL; previously deoxygenated for 30 min) and H2O (3.0 equiv) were added and the mixture was stirred for 5 min. Then, {PdBr[P(t-Bu)3]}2 (12.4 mg, 0.016 mmol) was added and the mixture was stirred at rt for 30 min. The resulting mixture was subsequently diluted with Et2O and filtered through silica using Et2O as the eluant. The filtrate was concentrated in vacuo and purified by column chromatography.
Hetarenes 19; General Procedure:[27]
In a glovebox, to a N2-filled dry reaction vessel containing the heterocyclic substrate (0.5 mmol) was added recrystallized, deoxygenated, and dried boronic acid (0.5 mmol), KF (87.0 mg, 1.5 mmol), and deoxygenated THF (1 mL). The mixture was stirred for 5 min and then {PdBr[P(t-Bu)3]}2 (4.1 mg, 0.005 mmol), THF (0.5 mL), and H2O (3.0 equiv) were added (THF and H2O were deoxygenated prior to use). After this, the mixture was stirred for an additional 30 min. Then, the mixture was quenched with Et2O and filtered through silica gel, the filtrate was concentrated, and the residue was purified by column chromatography.
Benzamides 20; General Procedure:[28]
CAUTION:
Carbon monoxide is extremely flammable and toxic, and exposure to higher concentrations can quickly lead to a coma.
To a CO-filled Schlenk tube, containing the catalyst complex (2.2 or 10 mol%), was added the aryl halide (0.45 mmol) and the amine (BnNH2 or piperidine; 4.6 mmol) in the stated solvent. The mixture was stirred in a preheated oil bath for the desired time. Then, the reaction was quenched with 1 M aq HCl (4.5 mL). The rubber septum was removed and the unreacted CO was vented into the fumehood. The crude product mixture was extracted with CH2Cl2 (2 × 5 mL), the extracts were filtered and concentrated, and the residue was purified by column chromatography (silica gel).
For the preparation of11C-radiolabeled benzamides, the procedure was as described above but with the use of11CO instead of CO.
α-Aryl Esters 22; General Procedure with tert-Butyl Propanoate:[29]
In a drybox, to a 4-mL, screw-capped vial containing Cy2NLi (0.243 g, 1.30 mmol) dissolved in toluene (2 mL), was slowly added the ester (1.10 mmol). After stirring at rt for 10 min, the soln was added to another 4-mL, screw-capped vial containing the aryl bromide (1.00 mmol) and catalyst {PdBr[P(t-Bu)3]}2. The vial was sealed with a cap containing a PTFE septum and removed from the drybox. After stirring at rt for 4 h, the mixture was diluted with Et2O (30 mL) and then washed with 0.1 M HCl (10 mL). The aqueous phase was extracted with Et2O (3 × 10 mL). To the combined organic layers was added sat. aq NaHCO3 (30 mL), and the aqueous layer was back-extracted with Et2O (3 × 10 mL). The combined organic layers were washed with H2O (30 mL), the aqueous layer was extracted with Et2O(3× 10 mL), and the combined organic layers were dried (MgSO4), and concentrated. The crude product was purified by flash column chromatography (silica gel, 2.5% EtOAc in hexanes).
tert-Butyl (4-tert-Butylphenyl)acetate (22, R1 = R2 = H; R3 = t-Bu; Ar1 = 4-t-BuC6H4); Typical Procedure on a Large Scale:[29]
To a N2-filled, 500-mL, three-necked round-bottomed flask equipped with a rubber septum, a glass stopper, and a stirrer-bar, was added Cy2NH (10.3 mL, 0.052 mol) dissolved in toluene (300 mL). The mixture was stirred for 10 min at 0 °C, and then a 2.5 Msoln of BuLi in hexane (20.7 mL, 0.052 mol) was added slowly to the cooled soln. Then, the mixture was stirred for 30 min at 0 °C. tert-Butyl acetate (5.93 mL, 0.044 mol) was added slowly over 20 min. The mixture was stirred for an additional 50 min at 0 °C. To another N2-filled, pear-shaped, 10-mL flask, containing {PdBr[P(t-Bu)3]}2 (0.062 g, 0.0790 mmol), were added 1-bromo-4-tert-butylbenzene (6.93 mL, 0.040 mol) and toluene (5 mL). The mixture was transferred via cannula to the round-bottomed flask containing the lithium enolate of tert-butyl acetate. Toluene (2 × 5 mL) was used to wash the pear-shaped flask, and the wash was transferred to the round-bottomed flask. The mixture was stirred for 4 h at rt, and then concentrated until the reaction volume was reduced to half by rotary evaporation. A sat. aq soln of NH4Cl (300 mL) was added, and the aqueous phase was washed with Et2O (5 × 200 mL). The combined organic layers were dried (MgSO4), filtered, concentrated, and purified; yield: 7.90 g (79.5%).
1.2.7.1.3 Variation 3: Cross-Coupling Reactions
Palladium-catalyzed cross-coupling reactions have been studied for about half a century. The typical mechanism involves oxidative addition and reductive elimination via two-electron transfer. Cross-coupling reactions involving alkyl halides, especially those with β-hydrogen atoms, are a challenge because of the slow rate of oxidative addition and the rapid rate of β-hydrogen elimination.[30] At the same time, cross-coupling reactions of the related alkylmetal compounds are also difficult because of the slow rate of transmetalation.[31] Recently, reactions that proceed via single-electron transfer processes have been developed, which have partially solved these problems. Generally, the radical properties of the reaction systems are supported by various radical-trapping experiments.
Cross coupling between 9-alkyl-9-borabicyclo[3.3.1]nonane (9-alkyl-9-BBN) derivatives and alkyl iodides has been achieved in the presence of a catalytic amount of tetrakis(triphenylphosphine)palladium(0) (▶ Scheme 17).[30] Primary iodides, even including iodomethane, deliver the products 23 in 45–71% yield; neopentyl iodide (1-iodo-2,2-dimethylpropane) also reacts successfully. However, reactions with secondary iodides are not successful. In terms of the boranes, various functionalized compounds are tolerated, including the presence of alkene, ester, and acetal groups.
Scheme 17 Cross-Coupling Reactions of 9-Alkyl-9-borabicyclo[3.3.1]nonane Derivatives with Alkyl Iodides[30]

R1 | R2 | Yield (%) | Ref |
Me | (CH2)10CO2Me | 71 | [30] |
(CH2)5Me | (CH2)7Me | 64 | [30] |
(CH2)5Me | ![]() | 58 | [30] |
(CH2)5Me | (CH2)10CO2Me | 54 | [30] |
(CH2)3CN | ![]() | 61 | [30] |
(CH2)3CO2Me | ![]() | 57 | [30] |
(CH2)9Me | (E)-CH=CHBu | 64 | [30] |
(CH2)9Me | Ph | 55 | [30] |
In 1987, reactions of polyfluoroalkyl iodides with organostannanes in a tetrakis(triphenylphosphine)palladium(0)-catalyzed Negishi coupling were reported (▶ Table 2).[32] The stannane can be allyl-, alkenyl-, and alkynyl-substituted. It is worthy to note that even trifluoroiodomethane leads to the desired product, albeit with lower yield (entry 3). Both the E– and Z-isomers of alkenylstannanes react with an iodide to afford only products with E configuration (entries 1, 2, 9, and 10). A possible mechanism is the addition of a polyfluoroalkyl radical to the alkenylstannane, followed by the elimination of tributyliodostannane; the palladium(0) species is thought to act as a radical initiator (▶ Scheme 18).
Entry | Starting Materials | Equiv of Iodide | Temp | Time (h) | Product | Yield (%) | Ref | |
Stannane | Alkyl Iodide | |||||||
1 | ![]() | F3C(CF2)3I | 2 | 70 °C | 4 | ![]() | 70 | [32] |
2 | ![]() | F3C(CF2)3I | 2 | 70 °C | 4 | ![]() | 70 | [32] |
3 | ![]() | CF3I | excess | 80 °C | 3 | ![]() | 11a | [32] |
4 | ![]() | F3C(CF2)5I | 1.2 | rt | 1 | ![]() | 100 | [32] |
5 | ![]() | F3C(CF2)5I | 3 | 70°C | 3 | ![]() | 64 | 32 |
6 | ![]() | F3C(CF2)3I | 2 | 70°C | 4 | ![]() | 68 | [32] |
7 | ![]() | F3C(CF2)5I | 2 | 70 °C | 6 | ![]() | 55 | [32] |
8 | ![]() | F3C(CF2)3I | 2 | 70 °C | 6 | ![]() | 60 | [32] |
9 | ![]() | F3CCH2I | 2 | 80 °C | 4 | ![]() | 38a | [32] |
10 | ![]() | F3CCH2I | 2 | 80 °C | 4 | ![]() | 35a | [32] |
a Benzene was used as solvent. |
Scheme 18 Possible Mechanism for the Introduction of a Polyfluoroalkyl Group to (E)-Tributyl(styryl)stannane[32]

Alkyl iodides can also participate in a palladium-catalyzed Heck-type reaction (▶ Table 3).[33] This intramolecular procedure results in five- or six-membered rings 24, which contain various functional groups. Preliminary investigations into the corresponding intermolecular reactions have also been undertaken (▶ Scheme 19). Three kinds of 1-aryl-2-cyclohexylethene are obtained in moderate yields. Radical-trapping reactions have helped to disclose the radical nature of this system (▶ Scheme 20). A mechanism was proposed, as shown in ▶ Scheme 21, whereby a palladium(0) species initiates the reaction via a single-electron transfer to generate carbon radical 25, which subsequently cyclizes onto the intramolecular alkene to provide another carbon radical 26. Then, radical 26 adds to palladium(I) and subsequent β-hydride elimination of the alkylpalladium(II) complex 27 leads to the product.
Substrate | Product(s) | Yield (%) | Ref |
![]() | ![]() | 80 | [33] |
![]() | ![]() | 70 | [33] |
![]() | ![]() | 73 (dr 83:17) | [33] |
![]() | ![]() | 74 | [33] |
![]() | ![]() | 70 | [33] |
![]() | ![]() | 62 (dr 58:42) | [33] |
![]() | ![]() | 66 (dr >95:5) | [33] |
R1 | Yield (%) | Ref |
Ph | 55 | [33] |
4-AcC6H4 | 60 | [33] |
4-(HOCH2)C6H4 | 51 | [33] |
Recently, intermolecular Heck reactions catalyzed by palladium that involve inactive alkyl halides have also been developed (▶ Scheme 22).[34] The method enables various primary and secondary alkyl iodides, including those with β-hydrogen atoms, to react with aryl-, hetaryl-, and electron-withdrawing-group-substituted alkenes. The observed reactivity of these reactions is consistent with a hybrid organometallic–radical pathway, which might be the reason for the avoidance of undesired dehydrohalogenation of the simple alkyl halide substrates. This method has been applied in the late-stage functionalization of a modified steroid to release 29 (▶ Scheme 23).[34] A radical clock reaction produces the cyclic substrate with no linear product observed (▶ Scheme 24); this result has helped to confirm the radical properties of this system. A mechanism has been proposed that is similar to the corresponding intermolecular reactions (▶ Scheme 25).
R1 | R2 | R3 | Yield (%) | Ratio (E/Z) | Ref |
Ph | H | Cy | 84 | E only | [34] |
4-F3CC6H4 | H | Cy | 82 | E only | [34] |
4-MeOC6H4 | H | Cy | 66 | E only | [34] |
2-pyridyl | H | Cy | 35 | E only | [34] |
CN | H | Cy | 70a | 29:71 | [34] |
CN | H | ![]() | 61a | 33:66 (dr 1:1) | [34] |
CN | Me | ![]() | 79a,b,c | 66:33 | [34] |
Ph | H | ![]() | 80 | 88:12 (dr 95:5) | [34] |
Ph | H | ![]() | 79 | E only | [34] |
Ph | H | ![]() | 42d,e | E only | [34] |
a K3PO4 (2.0 equiv) was used instead of Cy2 NMe. | |||||
b Pd(PPh3)4 (10 mol%) was used as the catalyst. | |||||
c Crotononitrile (3.0 equiv) was used. | |||||
d Reaction temperature was 130 °C. | |||||
e Yield determined by NMR. |
A tetrakis(triphenylphosphine)palladium(0)/1,1′-bis(diphenylphosphino)ferrocene system could also be used to realize the intermolecular Heck reaction of unactivated alkyl halides (▶ Scheme 26).[35] The desired alkenes are produced from various primary and secondary alkyl halides with improved E/Z selectivity (2:1 to 82:1); even a primary chloride reacts in 74% yield and with 4:1 (E/Z) selectivity. Secondary halides afford better E/Z selectivity than primary ones. Radical-trapping experiments have also been conducted with 2,2,6,6-tetramethylpiperidin-1-oxyl (TEMPO). The trapped product 31 is obtained (▶ Scheme 27) and a proposed mechanism is presented in ▶ Scheme 28.
R1 | X | Ar1 | Yield (%) | Ratio (E/Z) | Ref |
![]() | I | Ph | 75a | 10:1 | [35] |
![]() | I | Ph | 86 | 10:1 | [35] |
(CH2)3Ph | I | Ph | 75a | 4:1 | [35] |
(CH2)3NPhth | I | Ph | 76a | 17:1 | [35] |
Cy | I | 4-ClC6H4 | 82 | 38:1 | [35] |
Cy | I | 3-Tol | 70 | 30:1 | [35] |
CHEt2 | I | Ph | 80 | 38:1 | [35] |
![]() | Br | Ph | 64 | 82:1 | [35] |
(CH2)11 Me | Cl | Ph | 74a | 4:1 | [35] |
a Pd(dba)2 (10 mol%) and dppf (14 mol%) were used. |
Pd(PPh3)4 (mol%) | dppf (mol%) | Yield (%) | Ref |
10 | 14 | 20 | [35] |
20 | 28 | 41 | [35] |
50 | 70 | 100 | [35] |
In addition to the various cross-coupling reactions described above, the palladium-catalyzed C—H activation of hetarenes has also been achieved in reactions with unactivated alkyl halides (▶ Scheme 29).[36] The substrate scope is quite broad (86 examples; 50–96% yield), with common heterocycles generally tolerated. The use of secondary and tertiary iodides and bromides are the main focus, while only three examples employing primary halides are reported. Radical-trapping products have been isolated and identified, and based on these results and DFT studies a radical mechanism has been proposed (▶ Scheme 30). Single-electron transfer from [1,3-bis(diphenylphosphino)propane]palladium(0) [Pd(dppp)] to the alkyl halide produces an alkyl radical and PdI(X)(dppp); subsequent radical addition to the heterocycle generates a hetarene radical, which undergoes single-electron oxidation followed by deprotonation to finally deliver the product.
Ar1 | R1 | X | Yield (%) | Ref |
![]() | t-Bu | Br | 58a | [36] |
![]() | ![]() | Br | 62a | [36] |
![]() | ![]() | I | 61a,b | [36] |
![]() | 1-adamantyl | I | 80a | [36] |
![]() | Cy | I | 92c | [36] |
![]() | Cy | I | 98 | [36] |
![]() | Cy | I | 76 | [36] |
![]() | Cy | I | 80 | [36] |
![]() | Cy | I | 75d | [36] |
![]() | 1-adamantyl | I | 82a,e,f | [36] |
![]() | CH2Cy | I | 82a,e | [36] |
![]() | CH2Cy | I | 51a | [36] |
a NaI (2 equiv) was also added. | ||||
b dr 4:1. | ||||
c Ratio (C2/C4) 32:1. | ||||
d Ratio (mono-/dialkylation) 10:1. | ||||
e 10 mol% Pd(PPh3)4 and 14 mol% dppp were used. | ||||
f Ratio (C5/C3 alkylation) 23:1. |
Scheme 30 Proposed Mechanism of Palladium-Catalyzed C—H Activation of Hetarenes with Unactivated Alkyl Halides[36]

(E)-3,3,4,4,5,5,6,6,6-Nonafluoro-1-phenylhex-1-ene (▶ Table 2, Entry 1):[32]
To a tube containing a mixture of (E)-1-phenyl-2-(tributylstannyl)ethene (0.4 g, 1.0 mmol) and Pd(PPh3)4 (0.11 g, 0.10 mmol) in hexane (3.0 mL), was added a soln of F3C(CF2)3I (0.70 g, 2.0 mmol) in hexane (2.0 mL) dropwise. The mixture was stirred for 4 h at 70 °C. Et2O and sat. aq KF (10 mL) were added, and then the mixture was extracted with Et2O. The organic layer was dried (Na2SO4) and concentrated, and the residue was purified by chromatography (silica gel); yield: 0.23 g (70%).
Carbo- and Heterocycles 24; General Procedure:[33]
CAUTION:
Carbon monoxide is extremely flammable and toxic, and exposure to higher concentrations can quickly lead to a coma.
Alkyl iodide, Pd(PPh3)4 (10 mol%), 1,2,2,6,6-pentamethylpiperidine (PMP; 2.0 equiv), and benzene (0.5 M) (CAUTION: carcinogen) were added to a 22-mL Parr reactor in a glovebox. The reactor was removed from the glovebox, and then charged with CO (10 atm) twice. Then, the mixture was stirred for 24 h at 110 °C. The reactor was cooled and slowly depressurized, and then Et2O was added and the mixture was washed with 1 M HCl. The mixture was extracted with Et2O, and the organic layers were dried (MgSO4) and concentrated. The residue was dissolved in CH2Cl2 and treated with CuCl to remove Ph3P. The crude products were purified by flash column chromatography.
Alkenes 28; General Procedure:[34]
The alkyl iodide (0.87 mmol), alkene (1.31 mmol), PdCl2(dppf) (0.087 mmol), Cy2NMe (1.74 mmol), and PhCF3 (0.5 M) were added to a sealable tube in a glovebox. The mixture was removed from the glovebox and stirred for 14 h at 100 °C. Then, CH2Cl2 or Et2O was added, and the mixture was washed with 1 M HCl and extracted with CH2Cl2 or Et2O (3 × 10 mL). The organic layers were dried (MgSO4) and concentrated. The crude alkene was purified by flash chromatography. A similar process using Pd(PPh3)4 and K3PO4 was used to obtain compound 29.
Arylalkenes 30; General Procedure:[35]
Pd(PPh3)4 (28.9 mg, 0.025 mmol), dppf (19.4 mg, 0.035 mmol), and anhyd PhCF3 (2.0 mL) were added to a dry 10-mL reaction tube in an argon-filled glovebox. The alkyl halide (0.50 mmol), vinylarene (1.0 mmol), dodecane (GC standard; 20 μL), and Cy2NMe (146 mg, 0.75 mmol) were added after the mixture had been stirred for 30 min at rt. [For reactions of alkyl bromides, LiI (1.0 mmol, 134 mg, 2.0 equiv) was added to improve the yield by about 10%.] The mixture was stirred for 36 h at 110 °C, and then cooled. Et2O was added, and the mixture was passed through a short pad of silica gel. The filtrate was concentrated and the crude product was purified by flash chromatography (silica gel). The E/Z ratio was determined by GC for both the crude and isolated product.
Alkylhetarenes 32; General Procedure:[36]
Pd(PPh3)4 (29 mg, 0.025 mmol, 5 mol%), dppp (14 mg, 0.035 mmol, 7 mol%), and anhyd PhCF3 (3.0 mL) were added to a dry 10-mL Schlenk tube in an argon-filled glovebox. The mixture was stirred for 10 min at rt, and then the hetarene (60 mg, 0.5 mmol, 1 equiv), Cs2CO3 (326 mg, 1.0 mmol, 2 equiv), and alkyl iodide (1.0 mmol, 2 equiv) were added. For reactions of certain alkyl halides, NaI (2 equiv) was also added. The mixture was stirred at 110 °C until the hetarene was almost fully consumed. The mixture was concentrated and purified by flash chromatography (silica gel).
1.2.7.1.4 Variation 4: Carbonylation Reactions
Traditional palladium-catalyzed carbonylation reactions are initiated from aryl halides that participate in two-electron-transfer steps during the procedure. Recently, carbonylation involving alkyl halides in single-electron approaches has been developed. These strategies have further extended the scope of palladium-catalyzed coupling reactions. Diverse multicomponent reactions with cascade processes have also been efficiently completed, some of which are accelerated by light.
The Suzuki group has found that carbonylative cross-coupling reactions of 9-alkyl-9-borabicyclo[3.3.1]nonane (9-alkyl-9-BBN) derivatives with iodoalkanes could be achieved under carbon monoxide (1 atm) resulting in ketones 33 in moderate to good yield. The reactions are catalyzed by tetrakis(triphenylphosphine)palladium(0) and promoted by light (▶ Scheme 31).[37] The 9-alkyl-9-borabicyclo[3.3.1]nonane derivatives are generated in situ from terminal alkenes. The alkyl iodide can be primary, secondary, or tertiary. When 1-iodohex-5-ene is used, cyclic product 34 is formed in 60% yield, which gives evidence for the radical properties of this system.
Scheme 31 Carbonylative Cross-Coupling Reactions of 9-Alkyl-9-borabicyclo[3.3.1]nonane Derivatives with Alkyl Iodides[37]

R1 | R2 | Yielda (%) | Ref |
(CH2)5Me | (CH2)5Me | 67 | [37] |
(CH2)8CO2Me | Me | 76b | [37] |
(CH2)5Me | Cy | 65 | [37] |
(CH2)5Me | t-Bu | 69 | [37] |
![]() | ![]() | 73b | [37] |
CH2Me2CO2Me | ![]() | 65a,b,c | [37] |
(CH2)2OCH2Ph | (CH2)3CN | 70b | [37] |
a Yields determined by CLC. | |||
b Yield of isolated product. | |||
c Combined yield of the trans (54%) and cis (11%) isomers. |

Three-component cross-coupling reactions between alkyl iodides, carbon monoxide, and arylboronic acids under combined palladium/light conditions afford alkyl aryl ketones (▶ Scheme 32).[38] The reaction exhibits high substrate compatibility. Primary, secondary, and tertiary alkyl iodides all work well. In addition, arylboronic acids bearing electron-withdrawing or electron-donating groups and even 3-thienylboronic acid can be used. The reaction of (iodomethyl)cyclopropane under the standard conditions leads to ring-opened product 36; it is thought that a cyclopropylmethyl radical might be an important intermediate in the process. It is noteworthy that four-component reactions are also possible (▶ Scheme 33).
Scheme 32 Synthesis of Alkyl Aryl Ketones by Carbonylative Coupling of Alkyl Iodides and Arylboronic Acids[38]

R1 | Ar1 | Yield (%) | Ref |
(CH2)7Me | Ph | 92 | [38] |
(CH2)2O(CH2)3Me | Ph | 81 | [38] |
(CH2)6Cl | Ph | 83 | [38] |
(CH2)2Ph | Ph | 87 | [38] |
Cy | Ph | 81 | [38] |
(CH2)7Me | 2-naphthyl | 80 | [38] |
(CH2)7Me | 4-MeOC6H4 | 92 | [38] |
(CH2)7Me | 2-Tol | 95 | [38] |
Cy | 4-FC6H4 | 82 | [38] |
Cy | 3-thienyl | 82 | [38] |

When carbon monoxide is introduced into the system, the cross coupling between β-perfluoroalkyl-substituted alkyl halides and organostannanes affords the carbonylation products 37 (▶ Scheme 34).[39] Alkyl bromides exhibit lower reactivity than iodides. Allyl-, vinyl-, phenyl-, and alkynylstannanes all lead to the desired products, albeit in comparatively low yields in some cases.
R1 | R2 | X | Catalyst | Yield (%) | Ref |
(CF2)7CF3 | C=CPh | Br | Pd(PPh3)4 | 97 | [39] |
CF3 | Ph | I | PdCl2(PPh3)2 | 66 | [39] |
(CF2)5CF3 | Ph | I | PdCl2(PPh3)2 | 97 | [39] |
(CF2)7CF3 | CH=CHPh | I | PdCl2(PPh3)2 | 79 | [39] |
(CF2)7CF3 | CH2CH=CH2 | I | PdCl2(PPh3)2 | 51 | [39] |
When 4,5- or 5,6-unsaturated alkyl iodides are introduced to similar carbonylation conditions, cyclic products can be obtained. A palladium-catalyzed three-component cross-coupling reaction between tethered iodides, carbon monoxide (1 atm), and 9-alkyl- or 9-aryl-9-borabicyclo[3.3.1]nonane derivatives has been achieved to produce unsymmetrical ketones 38 in moderate to high yields through a cascade radical process (▶ Table 4).[40] The reaction rate is increased by irradiation using a tungsten lamp. The unsaturated iodide can be an alkene or alkyne. Annulation of iodocycloalkenes results in cis-fused bicyclic alkanes, though the stereochemistry of the carbonyl-containing side chains is not fixed. In the cyclization step, the formation of five-membered rings is favored over six-membered rings.
Table 4 Palladium-Catalyzed Three-Component Cross-Coupling Reaction of Iodides, Carbon Monoxide, and 9-Alkyl- or 9-Aryl-9-borabicyclo[3.3.1]nonane Derivatives[40]

Starting Materials | Product | Yield (%) | Ref | |
Alkyl Iodide | Borane | |||
![]() | ![]() | ![]() | 60 | [40] |
![]() | ![]() | ![]() | 73 | [40] |
![]() | ![]() | ![]() | 70 | [40] |
![]() | ![]() | ![]() | 74 | [40] |
![]() | ![]() | ![]() | 68 (dr 2:1) | [40] |
![]() | ![]() | ![]() | 77 (dr 2:1) | [40] |
The palladium-catalyzed carbon monoxide trapping cascades have been realized under irradiation using a xenon lamp to afford a series of five-membered cyclic keto esters and amides (▶ Table 5).[41] Generally, primary alkyl iodides result in moderate to good yields, while one primary bromide undergoes the transformation successfully (48% yield). When secondary iodides are used, the products are diastereomers. The selectivity is similar to related tin-catalyzed systems, so a mechanism based on a radical process has been proposed (▶ Scheme 35). Firstly, an alkyl radical 40 is generated under the mediation of palladium(0) and light. Radical 40 subsequently undergoes carbon monoxide trapping and 5-exo–trig acyl radical cyclization to result in a new alkyl radical 41, which in turn is trapped by another carbon monoxide molecule. This is followed by addition of the palladium(I) species to generate a palladium(II) species 42. Finally, the product is obtained via reductive elimination and esterification.
Substrates | Product(s) | Yield (%) | Ref | |
Alkyl Iodide | Alcohol or Amine | |||
![]() | Et2NH | ![]() | 61 | [41] |
![]() | MeOH | ![]() | 75 | [41] |
![]() | BnOH | ![]() | 83 | [41] |
![]() | EtOH | ![]() | 76 | [41] |
![]() | MeOH | ![]() | 68 | [41] |
![]() | MeOH | ![]() | 72 | [41] |
![]() | BuOH | ![]() | 82 | [41] |
![]() | MeOH | ![]() | 78 | [41] |
![]() | MeOH | ![]() | 74 | [41] |
![]() | MeOH | ![]() | 74 | [41] |
A cascade coupling between unactivated iodides, carbon monoxide, and alcohols or amines produces carboxylic acid esters 43 (▶ Table 6) or amides 44 and 45 (▶ Table 7), in a process that is catalyzed by palladium(0) complexes or decacarbonyldimanganese(0).[42] When amines are used, the use of palladium(0) complexes leads preferentially to dicarbonylation products 45 (keto amides), with a mixture of the amides 44 and keto amides 45 obtained (approximately 1:2 ratio). In contrast, the use of decacarbonyldimanganese(0) affords the single carbonylation products 44 selectively. Primary, secondary, and tertiary iodides are all suitable substrates for esterification and amidation systems. Ring-opening examples have been performed to further the understanding of the radical nature of the process; the cyclopropylmethyl radical has a high tendency to undergo rearrangement and then ring-opening[43] (▶ Table 6, entry 2 and Table 7, entry 2). In addition, the palladium(0)-catalyzed system has been applied in the synthesis of a natural product [(–)-hinokinin] and a natural product analogue (dihydrocapsaicin) (▶ Scheme 36).
Entry | Starting Materials | Base | Time (h) | Product | Yield (%) | Ref | |
Alkyl Iodide | Alcohol | ||||||
1 | ![]() | EtOH | Et3N/DMAP | 16 | ![]() | 87 | [42] |
2 | ![]() | BuOH | K2CO3 | 16 | ![]() | 83 | [42] |
3 | ![]() | BuOH | K2CO3 | 6.5 | ![]() | 83 | [42] |
Entry | Alkyl Iodide | Products | Yield (%) | Ref |
1 | ![]() | ![]() | 75 | [42] |
2 | ![]() | ![]() | 52 | [42] |
3 | ![]() | ![]() | 69 | [42] |
4 | ![]() | ![]() | 83 | [42] |
5 | ![]() | ![]() | 82 | [42] |
Three-component coupling reactions between alkyl iodides, carbon monoxide, and terminal alkynes have been achieved to deliver alkynyl ketones 46 under palladium/light-mediated conditions (▶ Scheme 37).[44] Primary, secondary, and tertiary alkyl iodides are all tolerated. The alkyne can be phenyl- or alkyl-substituted. Ring-opening and ring-closing experiments (▶ Scheme 38) have been performed to confirm the radical properties of this cascade reaction.
R1 | R2 | Yield (%) | Ref |
(CH2)7Me | Ph | 71 | [44] |
(CH2)7Me | (CH2)5Me | 88 | [44] |
(CH2)8Cl | Ph | 70 | [44] |
(CH2)3CO2Me | Ph | 63 | [44] |
CHMe(CH2)5Me | Ph | 65 | [44] |
(CH2)4OTBDMS | Ph | 53 | [44] |
CHMe(CH2)5Me | Ph | 65 | [44] |
(CH2)7Me | TMS | 58 | [44] |
1-adamantyl | Ph | 70 | [44] |
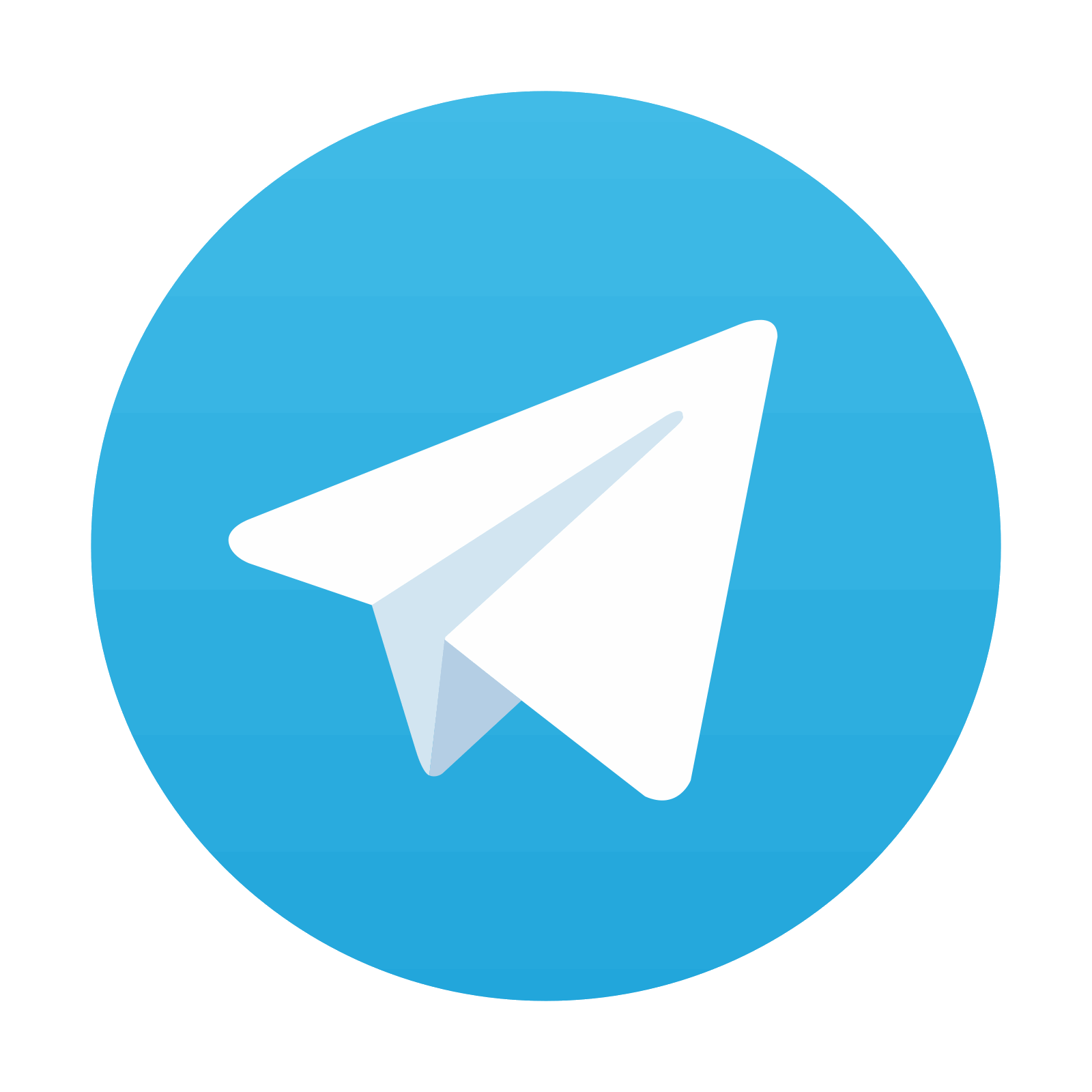
Stay updated, free articles. Join our Telegram channel
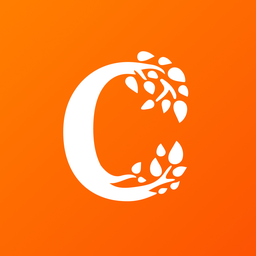
Full access? Get Clinical Tree
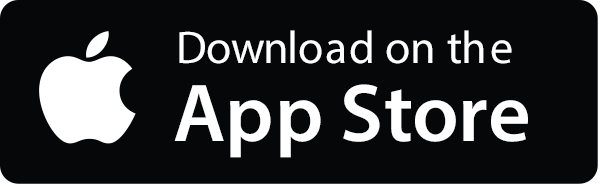
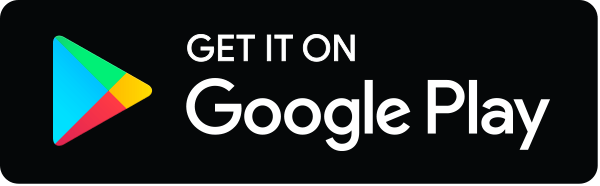