10 Cuiqing Liu and Qinghua Sun The lung is the organ primarily where the exchange of oxygen (O2) and carbon dioxide (CO2) between the atmosphere and vascular system takes place. It is also an entry and target organ for many inhaled toxins and toxicants, rendering the lung as one of the most vulnerable target organs resulting from exposure to commonly encountered toxicants. The chapter will mainly discuss: The primary function of the respiratory system is to obtain O2 for use by body’s cells and eliminate CO2 that cells produce. During this exchange the respiratory system also assumes an entry for “toxic” substances suspended in the inhaled air. Inhalant uptake is of importance for both occupational and environment exposures. It is essential to understand the anatomy and physiology of the respiratory system, which primarily include the respiratory tract and the lungs. The respiratory tract is the path that air takes when inhaled through the nose to the lungs, and can be divided into three main compartments, including the extrathoracic, tracheobronchial, and alveolar compartments. The extrathoracic compartment comprises the nose, mouth, nasopharynx, oropharynx, and larynx. Air enters through the nostrils of the nose and is partially filtered by the nose hairs, and it then flows into the nasal cavity. The nasal cavity is lined with epithelial tissue, containing blood vessels that help warm the air, and secrete mucous that further filters the air. The endothelial lining of the nasal cavity also contains tiny hair-like projections that are called cilia. The cilia serve to transport dust and other foreign particles, trapped in mucous, to the back of the nasal cavity and the pharynx. In addition, the sinuses (including maxillary sinuses, frontal sinuses, ethmoid sinuses, and sphenoid sinuses) are lined with soft, pink tissue called mucosa. In order to view these sinuses from different angles, Figure 10.1 shows a fontal view of the skull, while Figure 10.2 represents a sagittal view. Since the sinuses are connected to the nasopharyngeal airways through a number of small openings, inhaled air also enters the sinuses. When airborne irritants are inhaled the surfaces of sinus mucosa can be the first tissue to be irritated; this irritation may induce sinusitis and possibly lead subsequently to the growth of bacteria. Many factors can contribute to sinusitis, in addition to or in conjunction with inhaled toxins, such as allergic hypersensitivity, individual characteristics of the sinuses in each person, and climatic conditions. After passing through the nasal cavity, the air flows down the pharynx to the larynx. Figure 10.1 Frontal view of the skull, showing frontal, maxillary, and ethmoid sinuses. Source: Reproduced with permission from Fenn and Rahn (1964). Figure 10.2 Saggital view of the skull, showing nasal turbinates and sphenoid sinuses. Source: Reproduced with permission from Fenn and Rahn (1964). The tracheobronchial compartment includes the trachea, bronchi, and terminal bronchioles (Figure 10.3). The trachea, a tube surrounded with cartilaginous rings, carries air from the throat into the lung. It ranges from 20 to 25 mm in diameter and 10 to 16 cm in length in humans. The inner membrane of the trachea is also covered with cilia that catch particles in the dusts that can then be removed through coughing. The trachea connects the nasopharyngeal region with the two main bronchi, one entering left and the other entering right. The left bronchus is narrower, longer, and more horizontal than the right. Irregular rings of cartilage surround the bronchus, whose walls also consist of smooth muscle. Once inside the lung, the bronchi now divide up to 16–20 times and become the smaller airways or bronchioles. The bronchi themselves do not allow for the absorption of O2 or CO2 across their surfaces but are merely conducting airway tubes. The inner lining of the bronchi is covered by epithelial cells, which include ciliated cells, mucus-secreting goblet cells, serous cells, basal cells, and nonciliated bronchiolar epithelial cells. Here the synchronization of the ciliary beat is essential to the unidirectional movement of the mucus, which is transported by ciliary activity back toward the pharynx. It is by this mechanism that deposed particles can be removed from the tracheobronchial compartment and leave the body. Figure 10.3 Schematic representation of the subdivisions of the conducting airways and terminal respiratory units. The lung or alveolar compartment consists of the respiratory bronchioles, alveolar ducts, alveolar sacs, and alveoli, with a dramatic increase in the cumulative surface. In the bronchi proximal to the lung, very small air sacs or alveoli begin to appear. The bronchi in this region are known as respiratory bronchioles. The respiratory bronchioles lead to more than 10 million alveolar ducts, which in turn lead to more than 500 million alveoli, the termination of bronchioles. It is in these alveoli that gas exchange between the inhaled air and the blood circulatory system occurs. The surface of the alveoli is covered by two epithelial cell types, alveolar type I and alveolar type II cells. The alveolar type I cells are thin and cover about 90% of the alveolar surface. Alveolar type II cells are cuboidal and are more numerous, but cover only about 10% of the alveolar surface. These cells can secrete surfactants that line the inner surface of alveoli, which reduces the surface tension of the alveoli and is therefore very important for pulmonary mechanic movement and pulmonary function. The alveoli comprise approximately 80–90% of the total parenchymal lung volume with a total area for gas exchange of about 100 m2. In each alveolus, only a thin wall separates the blood in the capillary vessels from the inhaled air in the alveolus. This thin wall or respiratory membrane is a combination of the capillary endothelium, a basement membrane adjacent to the capillary, the space between the capillary and the alveolus (known as the interstitial space), a basement membrane adjacent to the alveolus, and the alveolar epithelium. Although the respiratory membrane consists of five layers, its thickness is only 0.4–2.5 μm. Therefore, CO2 and O2 readily cross this membrane by simple diffusion. As stated earlier, the large surface area of the lung produced by the alveolar ducts and alveoli is in continuous contact with the inhaled environmental atmosphere and the toxic inhalants present in it, rending the lungs as sites of action for adverse effects for volatile and particulate bound chemicals that might be present in the air. The main toxic inhalants are gas and airborne particles. Toxic gases refer to airborne chemicals that normally occur in the gaseous state and may have contact with the lungs or enter the body mainly by inhalation. Some well-known toxic gases include, but are not limited to, nitrogen dioxide (NO2), ozone (O3), sulfur dioxide (SO2), hydrogen sulfide (H2S), and chlorine (Cl2), and so on. The pattern of toxicity induced by these gases is in part determined by the sites of gas deposition in the respiratory tract. Water solubility is one critical factor in determining how deeply a given gas penetrates into the lung. High-soluble gases such as SO2 typically do not penetrate farther than the upper respiratory tract, and it is therefore relatively free of systemic toxicity. This is especially true in obligatory nose breathing species like the rat. In contrast, O3 and NO2 are relatively insoluble gases and so are not easily cleared by the upper respiratory tract and can penetrate deeply into the lung reaching the smallest airways and the alveoli where they are capable of eliciting toxic responses. In addition, very insoluble gases such as H2S efficiently pass through the respiratory tract, are taken up by the pulmonary blood supply, and are distributed throughout the body where toxicity and injury are induced. In addition, the condition of the alveolar–capillary membrane is important to gas diffusion. Once gas enters the alveolar space of the lung, it can cross the relatively permeable alveolar–capillary membrane complex and enter the pulmonary blood circulation. But poor health conditions in a patient might lead to the engorgement of the interstitial space with fluid, which would impair the diffusion of toxic chemicals across the alveolar–capillary membrane. Although preventing the free exchange of O2 and CO2 it may protect the affected individual from the toxic effects of the inhaled toxins that produce systemic toxicity once absorbed into the bloodstream. NOx comprises a group of gaseous chemicals that include nitric oxide (NO), NO2, nitrous oxide (N2O), dinitrogen dioxide (N2O2), nitrogen trioxide (NO3), dinitrogen trioxide (N2O3), dinitrogen tetraoxide (N2O4), and dinitrogen pentoxide (N2O5). Excluding N2O, the various NOx are interconvertible and many of them actually coexist in the atmosphere. However, from either an occupational, environmental exposure, or health perspective, the materials of most concern are NO2 and NO. A large amount of inhaled NO2 is removed from the respiratory tract, and absorption of up to 90% of the amount inhaled occurs in both human and laboratory animals. Absorption occurs along the entire tracheobronchial tree and within the respiratory (alveolar) region, but the major dose to tissue is delivered at the junction between the conducting and respiratory airways. Beyond this zone, a dramatic falloff in dose delivered to tissue occurs due to the rapid increase in lung surface area. Acute, high-level exposure to NO2, which may occur accidentally or in occupational settings, has clear health consequences in humans by producing emphysema-like changes and alterations in antimicrobial defenses. However, the extent to which adverse health effects may occur with long-term exposure to lower levels more relevant to ambient outdoor or indoor environments is yet to be resolved. Although the mechanism(s) underlying NO2-induced respiratory toxicology are not well known, data suggest the following: (i) NO2 exposure affects the nonspecific defense function clearance. Both tracheobronchial (due to mucociliary transport) and alveolar (due to macrophage activity) clearance studies support a graded response, whereby low NO2 levels accelerate and high levels retard clearance; most effects of NO2 on clearance seem to begin at higher than ambient levels. (ii) NO2 impairs resistance to infectious agents (bacteria and viruses) in animals exposed to levels as low as 0.5 ppm for 3 months. Data suggest that short-term repeated exposures may result in a reduction in counts of certain lymphocytes in the lungs or spleen, or a depression in antibody responsiveness to particular antigens. (iii) NO2 may enhance immune responsiveness by increasing the severity of pulmonary inflammation in sensitized lungs, and may play some role in the exacerbation of immune-mediated respiratory disease. (iv) Another explanation is that NO2 exposure may produce morphological alterations in the respiratory tract, especially in the centriacinar region, where the conducting and gas exchange airways meet. In the alveolar region, NO2 results in hypertrophy and hyperplasia of type I cells, followed by death and desquamation of these cells and proliferation of and replacement by type II cells, generating thickened the air–blood barrier. Bronchiolar response is characterized by hypertrophy and hyperplasia of epithelial cells, loss of secretory granules and surface protrusions in Clara cells, and loss of ciliated cells or cilia. Little is known about NO absorption and even less is known about its subsequent distribution. Because endogenous NO is involved in numerous physiological processes, the impact of inhaled, exogenous NO, especially at low concentrations, is often difficult to evaluate. In spite of any binding with hemoglobin, anoxia of O2-sensitive organs does not seem to occur, at least with NO levels not higher than 10 ppm. Additionally, methemoglobin, the product of NO and hemoglobin binding, is easily converted into ferrous hemoglobin by methemoglobin reductase. So, as long as the activity of methemoglobin reductase is maintained, the toxic effects for NO is much mitigated than NOx. O3 is the primary oxidant of concern in photochemical smog due to its inherent bioreactivity. It is a bluish, explosive, irritating, and highly toxic gas. Because of its poor water solubility, a substantial portion of O3 penetrates deeply into the lung although some 30% is scrubbed by the nasopharynx of humans. One of the biological actions of O3 is the reaction with unsaturated fatty acids. The strongly electrophilic nature of O3 enables it to attack carbon double bonds. In the presence of water, the ozonide undergoes hydrolysis with the formation of a carbonyl species and an intermediate zwitterion that ultimately decomposes into another carbonyl compound along with hydrogen peroxide. The ozonization of these fatty acids is essentially equivalent to lipid peroxidation and occurs primarily on biomembranes. Moreover, sulfhydryl compounds may scavenge O3 with the formation of disulfides and sulfonic acids. During the reactions of O3 with oxidizable compounds, reactive oxygen species (ROS) are produced, such as singlet oxygen, OH radicals, and hydrogen peroxide. These secondary reactive compounds may be responsible for subsequent reactions with amino acids and DNA. The primary sites of O3 injury are the epithelium of the nasal cavity, trachea and central acinar region. Ciliated cells in the nose and trachea, type I cells in alveolar ducts, and alveolar macrophages are the primary cell types injured. Classically, response of the respiratory system to O3 exposure can be characterized in terms of three stages: the initial response, proliferation and repair of the epithelium, and the response to continued exposure. Initial responses include injury and death of ciliated cells in the conducting airways and squamous epithelial cells in the parenchyma. The next stage is characterized by exfoliation of the epithelium and increased exudate. Inflammatory cells first appear in the interstitium and then in the epithelium, before they are present in the exudate. The third stage is marked by proliferation of the epithelium, concurrent with downregulation of intraluminal exudates. Significant numbers of inflammatory cells may still be found migrating through the epithelium at this stage, but within 7 days the acute inflammatory response is typically almost resolved. At this time, epithelial proliferation ceases, epithelium is often hyperplastic, and proliferation of matrix components is in progress. Then if O3 inhalation ceases, the assumption is that the affected compartments will revert to steady state within 7–10 days, which suggests that exposure during repair modifies this process. The effects of long-term exposure are characterized by continuous hyperplasia and low-grade chronic inflammation with exudative cells, as well as synthesis of collagen in the matrix. Although dose–response relationships to lasting effects on base-line lung function and structure are less firmly established, the magnitude of the response is dependent upon the inhaled dose of O3 and varies with the time course of exposure or the site of the airway tree. Current evidence supports a complex interaction between inhalation of environmental O3 and the pulmonary immune system. The biological response to O3 is dependent on genes of innate immunity including surface receptors, intracellular signaling molecules, and the production of downstream proinflammatory cytokines. Understanding the mechanisms that regulate the response to O3 can provide fundamental insight into gene and environment interactions and will broadly impact our understanding of inflammatory lung disease. SO2 is a colorless, highly water-soluble chemical irritant. It is absorbed quickly and almost entirely in the nose and upper airway during quiet breathing, but can be delivered to the lower airways during exercise. SO2 may quickly dissolve in the aqueous lining of the respiratory epithelium, forming acidic species that result in irritation of the mucous membranes, throat, and respiratory tract. Additionally, clinical studies have demonstrated that SO2 combined with exercise can cause significant bronchoconstriction in an exposure–response relationship. The chemical mechanisms underlying the bronchoconstrictor effects of SO2 were not well defined until now. Human and animal studies on SO2 have shown that it can affect various aspects of pulmonary defenses, such as mucociliary transport, alveolar clearance of deposited particles, and pulmonary macrophage function. H2S is a colorless, irritating, and highly toxic gas with a characteristic odor resembling rotten eggs, which is recognized at 0.025–1 ppm. H2S is primarily a systemic respiratory poison with cutaneous absorption being negligible. The most prevalent respiratory symptoms upon accidental exposure to H2S are dyspnea, sore throat, cough, and chest pain. Chronic inhalation of H2S may cause inflammation and dryness of the respiratory tract with rhinitis, pharyngitis, laryngitis, bronchitis, and pneumonia, the last of which may be related to the inhibitory effect of H2S on alveolar macrophages and their ability to inactivate bacteria. It also increases the respiratory rate by the stimulation of peripheral chemoceptors. In acute intoxications with high concentrations of H2S (>500 ppm), rapid olfactory paralysis occurs and death may ensue from respiratory failure with consequent asphyxia and cardiac failure. There are some other strong respiratory irritants that induce pulmonary damage after inhalation. Although the concentrations of respiratory irritants in occupational settings that produce toxic inhalation are generally very high and sustained only in incidents involving confined spaces, some of these same compounds are occasionally released in community exposures such as transportation accidents or pipeline leaks. Cl2 is a dense, acrid, pungent, greenish-yellow gas; it is a highly reactive oxidant gas and has higher solubility in water than NO2 and O3, two well-known environmental pollutants. Currently potential human exposure to chlorine inhalation occurs in a variety of settings in the workplace, as a result of inadvertent environmental releases, and even in the home due to household cleaning mishaps. When inhaled, Cl2 dissolves in the epithelial lung lining fluid, a thin layer of fluid covering the apical surfaces of airway and distal lung epithelial cells, and then reacts with biological molecules, such as low-molecular-weight antioxidants. Injury to airway and alveolar epithelium results from the chemical reaction products formed when Cl2 interacts with water (HCl and HOCl) and/or other reaction products, such as chloramines, that are formed from the reactions of these chlorinating species with biological molecules. Subsequent reactions may initiate self-propagating reactions and induce the production of inflammatory mediators compounding injury to pulmonary surfactants, ion channels, and components of lung epithelial and airway cells. Controlled human exposure data suggest that some subjects may be more responsive to the effects of chlorine gas; epidemiological data also indicate that certain subpopulations (e.g., smokers) may be at greater risk of adverse outcome after chlorine inhalation. Although these findings are intriguing, additional study is needed to better delineate the risk factors that predispose toward the development of long-term pulmonary sequelae following chlorine gas exposure. Hydrogen chloride (HCl) is another irritating chemical that is corrosive to any tissue with which it comes into contact. As it is highly soluble in water, following acute inhalation HCl is deposited in the nose and upper respiratory tract, causing irritation and ulceration, coughing, chest tightness, shortness of breathing, and choking. At higher concentrations, HCl may cause tachypnoea, swelling of the throat leading to suffocation, as well as pulmonary edema. Reactive airway dysfunction syndrome, a chemical-induced type of asthma, may also occur. Chronic inhalation exposure to HCl gas or mist may result in decreased pulmonary function, inflammation of the bronchi, and nasal ulceration. Table 10.1 presents the main gases/chemicals and some of their most relevant chemical and physical characteristics. Table 10.1 Toxic Gases and Characteristics Particulates mean a population of particles that remain suspended in the ambient air over time. In addition to the ability of the physical nature of particulate to induce lung injury if accumulated in the lungs, many particulates contain chemical and radionuclide agents that are also deposited in the respiratory tract in the form of solid particles or droplets. These particles are widely originated and present as aerosols, dust, fumes, smokes, mists, and smog. Dusts result from industrial processes such as sandblasting and grinding, and are identical to the compounds from which they originated. However, fume occurs with combustion or sublimation and derived from chemical change in compounds during processes such as welding. Smokes are produced during burning of organic materials, and mists are aerosols composed of water condensing on other particles. Smog is a conglomerate mixture of particles and gases that is prevalent in certain environments such as areas with mountains, plenty of sunlight, and periodic temperature inversions. The particle size is usually the critical factor both for the region of the respiratory tract in which a particle or an aerosol will be deposited and for the number of particles that will be deposited. Since most inhaled particles are irregular in shape, but not spherical, particles are frequently characterized in terms of equivalent spheres on the basis of equal mass, volume, or aerodynamic drag. Mass median aerodynamic diameter (MMAD) represents the diameter of a unit density sphere with the same terminal settling velocity as the particle, regardless of its size, shape, and density. If the number of particles is of primary interest, the count median diameter (CMD) is used to reflect the number of particles. Aerodynamic diameter is the proper measurement of particles that are deposited by impaction and sedimentation. Particle size is typically critical for very small particles, commonly deposited primarily by diffusion. However, it must be kept in mind that the size of particles may change during the course of traveling in the respiratory tract. Since the respiratory tract is highly humidified, materials that are hygroscopic, such as sodium chloride, sulfuric acid, and glycerol may absorb water and could be expected to increase in size as they descend in the respiratory system. Particles tend to deposit in the lung, which is determined by particle size, respiratory pattern, and regional characteristics of the respiratory tract. For example, very large particles are easy to be blocked out by nose hairs. The deposition of particles occurs primarily by interception, impaction, sedimentation, and diffusion (Brownian movement). Interception occurs when a particle comes close to a surface so that an edge of the particle contacts the airway surface. This process is important for the deposition of long particles, especially fibers, because it is usual for a fiber to be only 1 μm in diameter but 200 μm in length, leading to enhanced probability of contact with the respiratory tract surfaces. Particles suspended in air tend to continue to travel along the respiratory tract. In an airstream at an airway bifurcation, a particle may be impacted on the surface. At relatively symmetrical bifurcations, such as the human lung, the deposition rate is likely to be high for particles that move in the center of the airway. In an average adult, most particles larger than 10 μm in aerodynamic diameter are deposited in the nose or oral pharynx and cannot penetrate into the tissues distal to the larynx. Very fine particles (0.01 μm or smaller) are also trapped relatively efficiently in the upper airways by diffusion. Once particles penetrate beyond the upper airways, they are available to be deposited in the bronchial region and the deep-lying airways. Therefore, the alveolar region has significant deposition efficiencies for particles smaller than 5 μm and larger than 0.01 μm.
PULMONOTOXICITY: TOXIC EFFECTS IN THE LUNG
10.1 ANATOMY AND PHYSIOLOGY OF RESPIRATORY SYSTEM
Respiratory Tract
Lung
10.2 GENERAL PRINCIPLES OF INHALATION TOXICOLOGY
Gases
Nitrogen Oxides (NOx)
O3
SO2
H2S
Other Respiratory Irritants
Toxic Gas
Chemistry
Water Solubility
Site of Action
Exposure Settings
Toxic Effects in Respiratory System
Nitrogen dioxide (NO2)
Acid, oxidant
low
Entire airway and deep lung, especially the junction between the conducting and respiratory airways
Chemical industry, high-temperature combustion, nitric acid fumes, farms
Pulmonary edema; dyspnea and bronchiolitis obliterans; lung defense dysfunction
Ozone (O3)
Strong oxidizing agent
Low
Deep lung
Welding on aluminum, water treatment
Airway inflammation and permeability; decreased respiratory function
Sulfur dioxide (SO2)
Acid
High
Upper airway
Chemical industry, sulfur combustion, smelting
Bronchitis
Chlorine (Cl2)
Strong oxidizing agent
High
Airway and distal lung
Pulp mills, water disinfection, chemical industry, home cleaning
Bronchitis; persistent airways reactivity, chronic obstructive changes, BO
Hydrogen chloride (HCl)
Acid
high
Upper airway
Chemical industry, end uses
Bronchitis; pulmonary edema; decreased pulmonary function
Hydrogen sulfide (H2S)
Reducing agent
low
Entire airway and lung
Oilfields, refining, manure pits, sewer gas
Apnea; pulmonary edema; olfactory paralysis; pneumonia
Particles
Particle Size
Particle Deposition
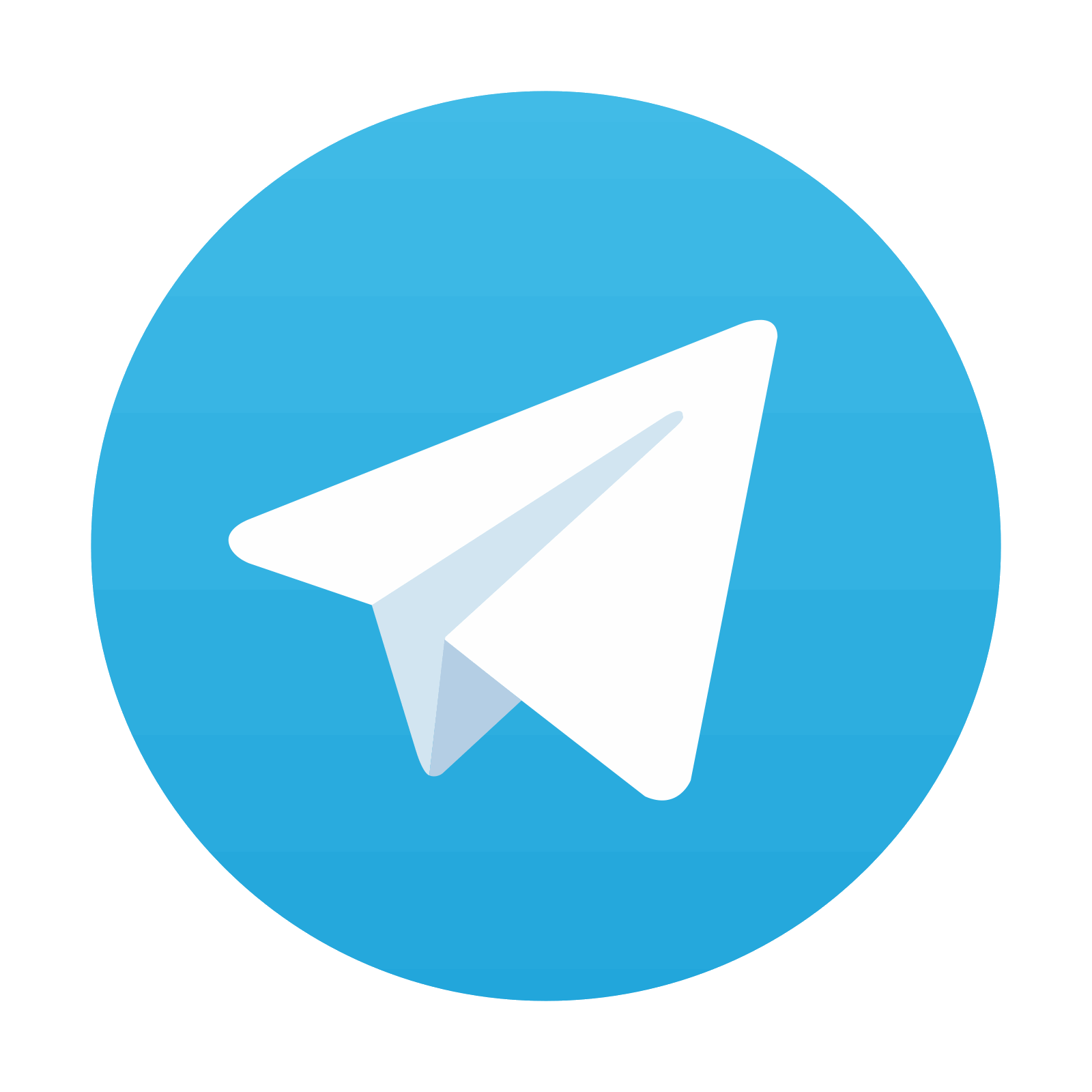
Stay updated, free articles. Join our Telegram channel
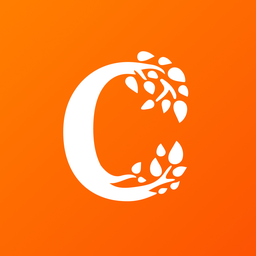
Full access? Get Clinical Tree
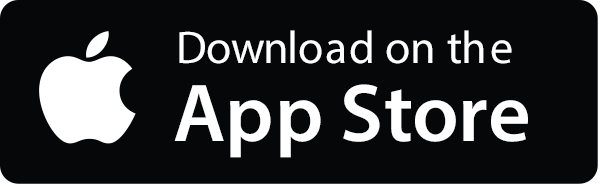
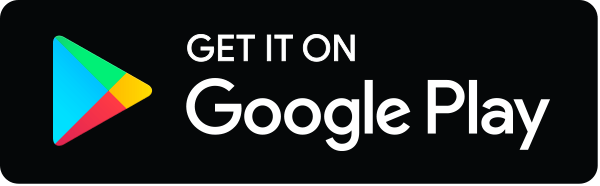