species thus providing a mechanism for proteinuria in conditions associated with high oxidative stress (9). In summary, the glycocalyx and the proteins bound to it likely form a size barrier and the polyanions (such as heparan sulfate) repel negatively charged albumin (10).
the filtration barrier. The GBM does provide a relatively small degree of size selective barrier to filtration. In an elegant experiment where cell-free glomerular skeleton, composed almost entirely of GBM, was used to study diffusion of Ficoll (a neutral substance), the contribution of GBM to diffusional resistance was found to be around 13% to 26% of total, increasing with size (14).
in the basal surface that has a circulating form—soluble uPAR (SuPAR) that is cleaved from the membrane. SuPAR has garnered interest in potentially being a marker of progressive decline in kidney function (16). The slit diaphragm, which is the space between adjacent foot processes, is a zipper-like structure formed by nephrin, neph1-3, p-cadherin, and FAT (17). Nephrin gene mutation is associated with congenital nephrotic syndrome of the Finnish type (18). Podocin is another important slit diaphragm protein that interacts with nephrin. Podocin plays a crucial role in maintaining the filtration barrier. Mutation of podocin is associated with familial steroid-resistant nephrotic syndromes (19).
can cause redistribution of F-actin fibers in the cytoskeleton, resulting in damaged podocytes (20). Hyperglycemia-induced glycated albumin could potentially also be involved in podocyte injury and damage (10). In a recent mice experiment, when endothelium-specific hyaluron synthase was missing, the endothelial glycocalyx later was lost, the mice developed albuminuria, and within weeks developed podocyte injury and glomerulosclerosis (21). Studies of drugs that stabilize the glycocalyx layer, such as sulodexide, however, have failed to demonstrate beneficial effects in progression of diabetic kidney disease (22, 23).
curves bearing open symbols represent the clearance of dextrans by the normal human kidney. As the radius of dextrans increases, their clearance relative to inulin, and therefore to water, decreases.
reported that inhibition of NO caused both glomerular hypertension and proteinuria in normal rats (51). It is not known at this time whether NO modulates proteinuria in nephrotic syndrome in patients.
of lower molecular weight, such as β2 microglobulin (MW 11.6 kDa) and α1 microglobulin (MW 31 kDa). Albumin with a molecular weight of 69 kDa is only modestly increased in tubular proteinuria, when the glomerular filtration barrier is intact. In its most severe form, proximal tubular dysfunction is characterized by the “Fanconi syndrome” where there is an inability to reabsorb glucose, amino acids, uric acid, phosphate, bicarbonate, and other normal components of proximal tubular fluid in addition to proteins. As a consequence, the Fanconi syndrome causes a nonanion gap metabolic acidosis, hypouricemia, hypophosphatemia, aminoaciduria, and glycosuria in addition to proteinuria. In addition, high urinary concentrations of molecules such as parathyroid hormone, insulin, insulin-like growth hormone (IGF-1) and chemokine monocyte chemoattractant protein-1 (MCP-1) were found in patients with Fanconi syndrome, which may potentially be involved in progressive interstitial fibrosis and renal failure (53, 54). Urinary retinol binding protein (RBP) might also be valuable in diagnosis of tubular proteinuria (55) and urinary RBP: creatinine ratio has been suggested by some as a useful screening test for tubular proteinuria (56, 57). The Fanconi syndrome may result from inherited metabolic disorders such as Dent’s disease, cadmium exposure, light chain-associated diseases such as myeloma, amyloidosis, etc. In clinical practice, probably the most commonly encountered setting for Fanconi syndrome is medications such as tenofovir (56). Early detection of proximal tubular defects by medications such as tenofovir is important as withdrawal of such agents have been shown to have beneficial effects on estimated glomerular filtration rate (GFR) (58). Table 14-1 lists some causes of tubular proteinuria.
Table 14-1 Disorders Causing Impaired Renal Reabsorption of Filtered Proteins at Normal Filtered Loads | ||||||||||||||||||||||||||||||||
---|---|---|---|---|---|---|---|---|---|---|---|---|---|---|---|---|---|---|---|---|---|---|---|---|---|---|---|---|---|---|---|---|
|
a patient with this disorder. There is a large quantity of light chain protein in the urine (a so-called “spike”). Some myeloma light chain proteins are quite nephrotoxic, depending on their isoelectric points (pKi) and other factors. Myeloma light chain proteins with a pKi of around 5 generally are most toxic, in part because of their reduced solubility in the acid milieu of the renal papilla (60). Benign causes of overflow proteinuria include myoglobinuria in patients with rhabdomyolysis (61).
Table 14-2 Causes of Overflow Proteinuria | ||||
---|---|---|---|---|
|
blood using the benzidine test, even in the absence of red blood cells. It is important to identify this entity for two reasons. Most significantly, myoglobinuria is an important cause of acute kidney injury (76, 77, 78). The mechanism for the acute kidney injury caused by myoglobinuria is probably multifactorial, including intense renal vasoconstriction, tubular obstruction, and, most importantly, tubular injury. Iron contained in the heme moiety of myoglobin causes direct tubular damage by acting as a Fenton (free radical) reagent (79). Therapies suggested to mitigate or attenuate myoglobin-induced acute kidney injury include vigorous parenteral fluid administration to help accelerate renal clearance of myoglobin and the addition of sodium bicarbonate and mannitol to the parenteral fluids. Alkalinizing the urine with the addition of sodium bicarbonate to parenteral fluids may help prevent the heme moiety separating from the globin component of myoglobin and thus prevent iron-induced tubular damage (80). The addition of mannitol has a number of theoretical benefits to attenuate acute kidney injury; mannitol is an osmotic diuretic and would accelerate urinary excretion of myoglobin, and it increases renal blood flow and is a scavenger of free radicals (81). However, the routine use of mannitol is not recommend in this setting given the questionable overall clinical benefit (82).
composition that also characterize the nephrotic syndrome? What other proteins are either decreased by loss or inappropriately increased in response to changes in plasma composition that contribute to morbidity? Why are urinary protein losses resistant to replacement by dietary protein augmentation and what are the effects of dietary protein augmentation both on plasma protein composition and on renal function? These are questions that are approached in the ensuing sections.
Table 14-3 Causes of Glomerular Proteinuria | ||||||||||||||
---|---|---|---|---|---|---|---|---|---|---|---|---|---|---|
|
data are available demonstrating the effectiveness of these diets in restoring protein pools. Increased dietary protein intake in fact fails to increase either albumin concentration or body albumin pools in patients with the nephrotic syndrome (142) (Fig. 14-9) or animals with experimentally induced nephrotic syndrome (123, 135, 143, 144). Instead, much of the ingested protein is catabolized rather than used for net protein synthesis, and dietary protein augmentation also increases renal albumin clearance, causing any increased albumin that is synthesized to be lost in the urine. Furthermore, the increased albumin synthesis that results from dietary protein augmentation is accompanied by an increased rate of high-molecular-weight proteins, fibrinogen and of α2 macroglobulin (145, 146) that may play a role in the coagulopathy associated with the nephrotic syndrome, as will be discussed subsequently. In addition, dietary protein exerts an effect on the kidney, causing a reversible increase in glomerular permeability to large macromolecules (147), so most of the additional albumin synthesized is lost in the urine. Figure 14-10 shows the effect of diets containing either 2 or 0.6 g/kg of protein on the renal clearance of neutral dextrans when fed to nephrotic patients. It can be seen clearly that patients clear high-molecular-weight dextrans more easily when fed a high-protein diet. Thus, a change in dietary protein may alter the permselectivity characteristics of the glomerular filtration barrier in these patients increasing the renal clearance of albumin so that the net effect may be one of decreasing albumin stores (95, 134).
compared to less severe restriction (150). It should be noted that the effect of dietary protein on albumin homeostasis in nephrotic patients has compared a usual protein intake (approximately 1.2 g/kg) to a modest level of protein restriction (0.8 g/kg), a value that more closely approaches the control group in studies of the effect of protein restriction on loss of renal function (151). Continued maintenance of a high-protein diet may have the consequence of causing permanent rather than transient changes in the kidney and accelerate the progression of renal diseases (148, 149, 152). Increased filtration and tubular metabolism of plasma proteins may increase the injurious effect of high protein intake (153).
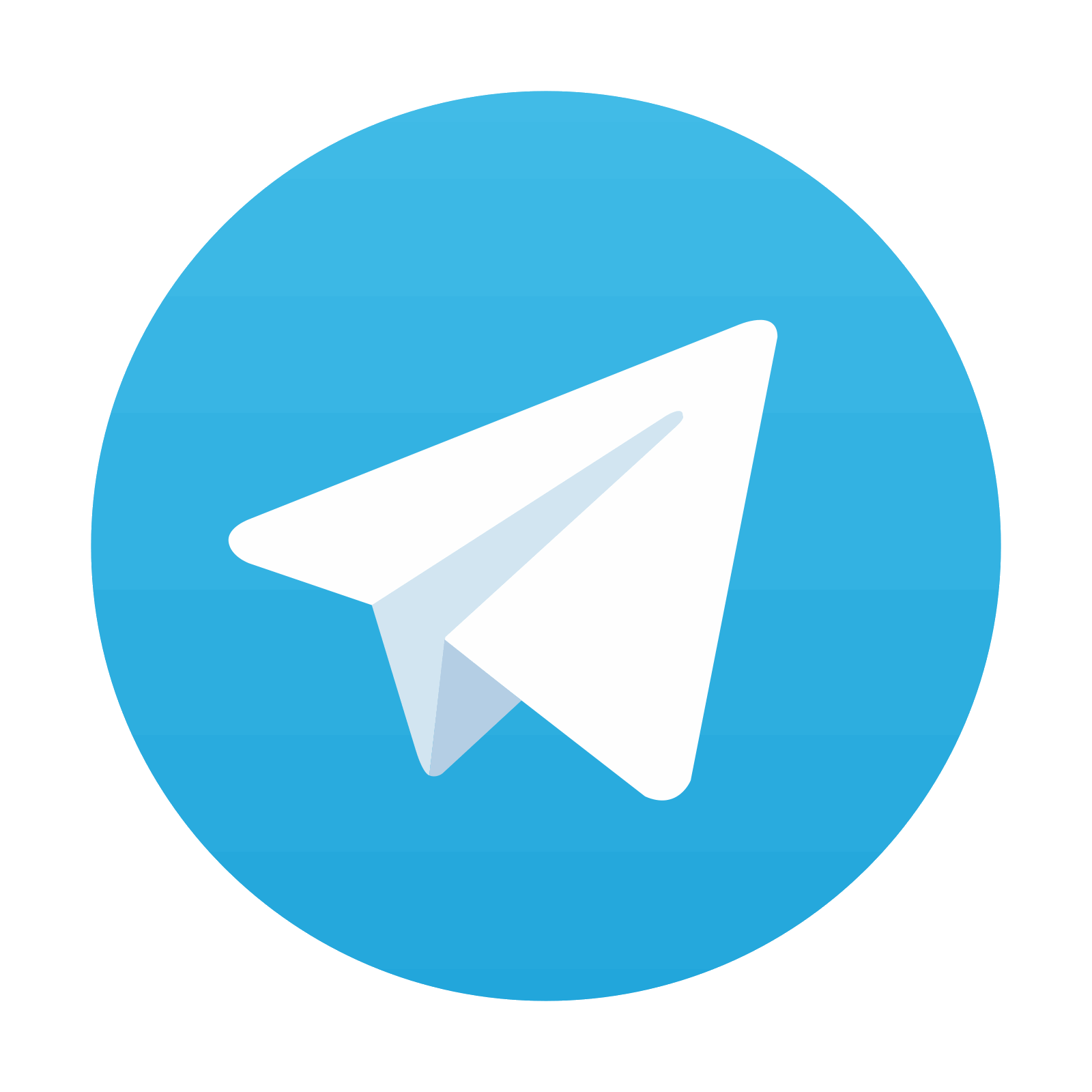
Stay updated, free articles. Join our Telegram channel
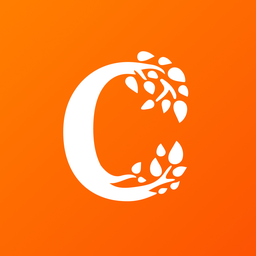
Full access? Get Clinical Tree
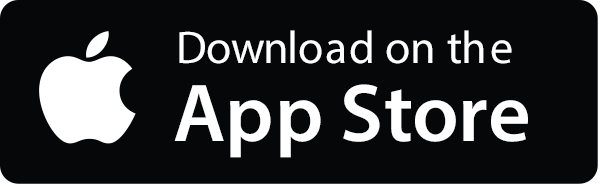
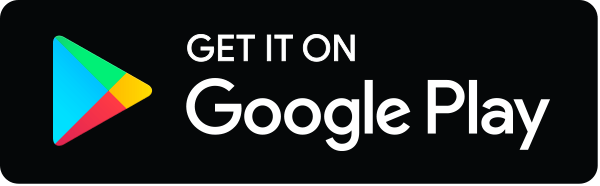
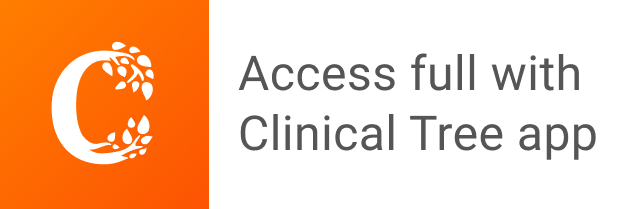