Disorders of the Renin-Angiotensin-Aldosterone System
John M. Carson
Matthew K. Abramowitz
Manish P. Ponda
Thomas H. Hostetter
The renin-angiotensin-aldosterone system (RAAS) has been conserved through evolution and is present in all vertebrates (1). It has long been known to play a central role in the regulation of blood pressure and renal sodium and water excretion. The classic, linear description of the RAAS begins with the conversion of the liver-derived glycoprotein angiotensinogen into the inactive angiotensin (Ang) I by renin, a protease secreted by the juxtaglomerular (JG) cells of the kidney. Ang I is then cleaved by angiotensin-converting enzyme (ACE) into Ang II, which has a diverse range of physiologic effects, including acting as an aldosterone secretagogue in the adrenal cortex. Aldosterone stimulates sodium reabsorption in the distal nephron. The system forms a feedback loop whereby secretion of the main effectors is affected by other members of the RAAS cascade and by other mediators.
The understanding of this system has become increasingly complex over the past two decades with the identification of new Ang receptors, a (pro)renin receptor and additional Ang peptides (Fig. 8-1). Numerous disparate physiologic and pathophysiologic effects have been attributed to the angiotensins and to aldosterone. The notion of the RAAS as an endocrine system has been expanded to include paracrine and autocrine effects with local production of Ang II at the tissue level. This chapter begins with an overview of each component of the RAAS, followed by a discussion of the pathophysiologic importance of each. We then discuss RAAS blockade and its importance in the treatment of individuals with chronic kidney disease (CKD).
Angiotensinogen
Angiotensinogen is a 55- to 60-kDa serum glycoprotein that serves as the precursor for all Ang peptides. It is the sole circulating renin substrate; cleavage of a leucine-valine bond produces the decapeptide Ang I. The variability in molecular size of angiotensinogen is attributed to different patterns of glycosylation, as the angiotensinogen gene encodes only a single protein product (2). A high-molecular-weight variant has also been identified that is normally present at low levels. It exists as a greater fraction of total plasma angiotensinogen during the third trimester of pregnancy and is associated with hypertension and preeclampsia in pregnant women (3).
Angiotensinogen is primarily synthesized and released by the liver. Hepatic production may be increased by a number of factors. Angiotensinogen is an acute-phase reactant, and its synthesis is stimulated by stressors such as infection. Glucocorticoids, estrogens, and thyroxine increase angiotensinogen production by the liver (4). Ang II stimulates angiotensinogen synthesis in a positive feedback loop, leading to greater presence of the proximal substrate of the renin-angiotensin system (RAS) at times of increased Ang II production (5).
There is evidence of local angiotensinogen production in multiple organ systems as well. Angiotensinogen mRNA expression has been demonstrated in the kidney, heart, vascular tissues, adrenal gland, central nervous system, fat, and leukocytes (2, 6). Within the kidney, angiotensinogen mRNA is most abundant in the cortex, especially in the proximal tubule, but it is also present in the glomerulus and medulla (7).
Renin
Renin is a 37- to 40-kDa aspartyl protease with high specificity for angiotensinogen, its only known substrate. Translation of renin mRNA yields the inactive precursor preprorenin, which is converted to prorenin by the removal of a 23-amino acid signal peptide from the carboxyl terminus during insertion into the endoplasmic reticulum (8). Prorenin is a proenzyme that may be rapidly and directly secreted in the intact form or packaged into immature granules and processed into the active renin. Both prorenin and renin are secreted by the JG cells of the kidney but the former is the major circulating form as its plasma concentration is 10-fold higher than that of renin (9). Some have speculated that prorenin may be converted to renin in the circulation or locally in tissues, and prorenin-activating enzymes have been found in vascular endothelial cells and neutrophils (10). Renin mRNA has also been demonstrated in multiple organs other than the kidney, including the brain, liver, lung, submandibular gland, prostate, testis, ovary, spleen, pituitary, and thymus (11). Nevertheless, extrarenal production of renin has not been clearly demonstrated, and extrarenal sites that express the renin gene secrete prorenin, not renin (12).
A functional renin receptor, called the (pro)renin receptor, possesses both renin- and prorenin-specific binding. It has been localized to the mesangium in glomeruli, smooth muscle cells in renal and coronary
arteries, placenta, brain, and liver (13). Binding of renin and prorenin increases the catalytic efficiency of angiotensinogen cleavage and also induces intracellular signaling via activation of the mitogen-activated protein (MAP) kinases ERK1 and ERK2 (13). Through activation of this pathway, (pro)renin receptor binding increases the expression of profibrotic molecules including transforming growth factor (TGF)-β1, plasminogen activator inhibitor-1 (PAI-1), fibronectin, and collagen I (14, 15, 16), as well as the proinflammatory mediators interleukin-1β (IL-1β), cyclooxygenase-2, and tumor necrosis factor-α (TNF-α) (17, 18). These studies suggest a functional role for prorenin via nonproteolytic activation induced by receptor binding. They also demonstrate the Ang II-independent, receptor-mediated effects of renin. As such, renin itself may contribute to the progression of CKD by promoting fibrosis and inflammation.
arteries, placenta, brain, and liver (13). Binding of renin and prorenin increases the catalytic efficiency of angiotensinogen cleavage and also induces intracellular signaling via activation of the mitogen-activated protein (MAP) kinases ERK1 and ERK2 (13). Through activation of this pathway, (pro)renin receptor binding increases the expression of profibrotic molecules including transforming growth factor (TGF)-β1, plasminogen activator inhibitor-1 (PAI-1), fibronectin, and collagen I (14, 15, 16), as well as the proinflammatory mediators interleukin-1β (IL-1β), cyclooxygenase-2, and tumor necrosis factor-α (TNF-α) (17, 18). These studies suggest a functional role for prorenin via nonproteolytic activation induced by receptor binding. They also demonstrate the Ang II-independent, receptor-mediated effects of renin. As such, renin itself may contribute to the progression of CKD by promoting fibrosis and inflammation.
Regulation of Renin Secretion
The majority of renin production occurs in the JG cells on the afferent arterioles of the kidney. In normal subjects, sodium intake is the main determinant of renin secretion. Low sodium intake, resulting in reduced extracellular volume, stimulates renin release. Conversely, high sodium intake inhibits renin secretion through extracellular volume expansion. Several mechanisms, which primarily sense volume changes, regulate renin production and secretion (Table 8-1).
RENAL BARORECEPTORS
The JG cells, of myoepithelioid origin, sense changes in renal perfusion pressure through changes in stretch of the afferent arteriolar wall. JG cells increase renin secretion in response to decreased stretch with reduced perfusion pressure. Conversely, renin secretion is inhibited in response to increased pressure or stretch within the afferent arteriole. The coupling of perfusion pressure with renin release is likely mediated by changes in cytosolic calcium concentration related to JG cell stretch (19).
MACULA DENSA
Renin secretion is also regulated by the macula densa, an area of closely packed specialized tubular cells in the thick ascending limb of the loop of Henle. The composition of tubule fluid delivered to the macula densa regulates renin release via an ion-sensing mechanism that is independent of volume. Infusion of sodium chloride produces a rapid decline in plasma renin activity (PRA) that is not seen with comparable volume expansion with a dextran-containing solution (20). While this effect was initially thought to be sodium dependent, further studies demonstrated the importance of the chloride concentration of tubular fluid. PRA is not suppressed
by infusion of non-chloride-containing sodium solutions, but it is suppressed by non-sodium-containing chloride solutions (21). Thus, renin inhibition is thought to be related to the magnitude of chloride absorption in the macula densa. Isolated perfusion of these structures has shown that lower sodium chloride in the lumen of the macula densa stimulates renin secretion. This depends on salt entry into macula densa cells via the Na+/K+/2Cl– cotransporter (22). As the tubular cells of the macula densa are not in direct contact with the afferent arteriolar JG cells, additional mechanisms involving second messenger signaling and paracrine factors have been postulated. Adenosine inhibits renin secretion and has been proposed as a mediator of macula densa-regulated renin release (23).
by infusion of non-chloride-containing sodium solutions, but it is suppressed by non-sodium-containing chloride solutions (21). Thus, renin inhibition is thought to be related to the magnitude of chloride absorption in the macula densa. Isolated perfusion of these structures has shown that lower sodium chloride in the lumen of the macula densa stimulates renin secretion. This depends on salt entry into macula densa cells via the Na+/K+/2Cl– cotransporter (22). As the tubular cells of the macula densa are not in direct contact with the afferent arteriolar JG cells, additional mechanisms involving second messenger signaling and paracrine factors have been postulated. Adenosine inhibits renin secretion and has been proposed as a mediator of macula densa-regulated renin release (23).
Table 8-1 Factors Regulating Renin Secretion | ||||||||||||||||||||||
---|---|---|---|---|---|---|---|---|---|---|---|---|---|---|---|---|---|---|---|---|---|---|
|
NEURAL MECHANISMS
Neural mechanisms modulate renin release, primarily via the sympathetic nervous system. This appears to be mediated by β-adrenergic receptors, based on several lines of evidence. The JG apparatus (JGA) is densely innervated with sympathetic nerves, and β1-adrenoreceptors have been localized to the JGA and glomerulus (24, 25, 26). β-adrenergic agonists and increased renal sympathetic nerve activity stimulate renin release, while β-adrenergic antagonists inhibit this effect and reduce renin secretion (23). The neural modulation of renin release appears to involve the adenylyl cyclase signaling pathway via cyclic adenosine monophosphate (cAMP).
ENDOCRINE AND PARACRINE MECHANISMS
Multiple circulating factors regulate the release of renin. The most important of these is Ang II, which affects renin release through a negative feedback loop that is independent of volume or tubular transport processes. Higher Ang II levels directly inhibit renin secretion by regulating renin gene expression in the afferent arteriole (27, 28). ACE inhibition increases renal renin mRNA expression in animal models (27, 29) and increases PRA in humans, at least in part, by interrupting the inhibitory feedback of Ang II (30).
Numerous other hormonal influences affect renin levels via both endocrine and paracrine mechanisms (12). Activators of adenylyl cyclase, by increasing cAMP levels, stimulate renin secretion. These include prostaglandin E2, prostacyclin, dopamine, and glucagon. Nitric oxide indirectly increases cAMP levels and thus also promotes renin release. Natriuretic hormones, such as atrial natriuretic peptide (ANP), inhibit renin secretion via guanylyl cyclase activation. Calcium has been known to suppress renin release from JG cells. This effect is mediated by calcium-dependent inhibition of adenylyl cyclase (31). As such, calcium-liberating hormones such as endothelin, vasopressin, and adenosine block renin secretion as well. Vitamin D appears to negatively regulate renin expression via a calcium-independent mechanism (32).
Angiotensin-Converting Enzyme
ACE is a zinc-containing metalloprotease with a molecular weight of approximately 200 kDa. It is a type I ectoprotein with a long ectodomain that includes the enzymatic active site, a transmembrane domain, and a short cytoplasmic domain (33). ACE cleaves the two C-terminal amino acids (His-Leu) from Ang I to form the octapeptide Ang II. The conversion of Ang I to Ang II occurs rapidly throughout the vasculature. ACE is located on the surface of endothelial cells in many vascular beds, including the kidney. Because the pulmonary vasculature is the main site of ACE synthesis, a single pass through the lung produces nearly complete conversion of Ang I to Ang II (34).
In contrast to the substrate specificity of renin, multiple small peptides are hydrolyzed by ACE, including bradykinin, enkephalins, substance P, and luteinizing hormone-releasing hormone (35, 36, 37, 38, 39). Thus, in addition to generating the potent vasoconstrictor Ang II, ACE degrades the vasodilator bradykinin into inactive fragments. This underscores the multiple biologic pathways in which ACE may play an important role. The plasma concentration of ACE may be affected by a number of disease states, including hypothyroidism, diabetes mellitus, sarcoidosis, and other granulomatous diseases (40, 41, 42). Yet despite significant variation in ACE levels even among healthy subjects, a significant association between levels of the enzyme and the risk of hypertension has not been identified (43, 44).
The human ACE gene has been localized to chromosome 17 (43). There are two isoforms of ACE, a larger somatic ACE (sACE) and a smaller germinal ACE (gACE). gACE is expressed in sperm cells, likely regulated by androgens, and plays a role in male fertility (45). A soluble form of ACE, formed by cleavage of the ectodomain
of sACE, exists in plasma. While its function is not fully understood, soluble ACE is enzymatically active and appears to play a role in renal development and function, but not blood pressure (46).
of sACE, exists in plasma. While its function is not fully understood, soluble ACE is enzymatically active and appears to play a role in renal development and function, but not blood pressure (46).
Within the kidney, ACE is expressed in the brush border of the proximal tubule and on vascular endothelial cells (47, 48). Postulated functions for ACE located on the brush border membrane of the proximal tubule include cleavage of filtered peptides for subsequent uptake by epithelial cells and local production of Ang II within proximal tubule fluid to facilitate reabsorption (48).
ACE expression in the vasculature is not limited to the endothelium. Macrophages and other inflammatory cells are significant sources of tissue ACE in human atherosclerotic plaques (49). An identified insertion/deletion (I/D) polymorphism of a 287-base-pair DNA fragment within the ACE gene is associated with clinical outcomes. Specifically, the deletion polymorphism is associated with higher ACE levels (44) and with increased risk of microalbuminuria, retinopathy, and left ventricular hypertrophy in hypertensive subjects (50). The D allele is also associated with kidney disease in individuals with hypertension (51) and diabetes (52). Patients with diabetic nephropathy homozygous for the D allele compared to homozygosity for the I allele may benefit less from the renoprotective effects of ACE inhibitors (53).
Angiotensins
Ang II is a highly potent vasoconstrictor with multiple actions discussed in more detail later. Ang II is formed from cleavage of the C-terminal dipeptide of Ang I by ACE. Alternative pathways for Ang II generation have been demonstrated, but their physiologic importance is unclear. Tonins, cathepsins, and kallikreins can form Ang I or Ang II directly from angiotensinogen (54). Recent evidence suggests that chymase-dependent pathways may be important for Ang II generation within cardiovascular tissues (55). The half-life of Ang II in the circulation is approximately 1 to 2 minutes. It is hydrolyzed by aminopeptidase A to form Ang III, the heptapeptide Ang 2-8 (56). Ang II is also converted to Ang 1-7 and Ang A.
Ang III is a less potent peripheral vasoconstrictor than Ang II (57, 58), with a much shorter plasma half-life (59). This is likely due to its greater affinity for angiotensin type 1 (AT1) receptors than angiotensin type 2 (AT2) receptors. There is no known receptor specific to Ang III. In AT1 receptor-blocked rats, Ang III produces natriuresis and vasodilation (60). In the 1970s, Ang III was shown to stimulate aldosterone release from the adrenal zona glomerulosa (61). It also appears to be an important mediator of the RAS in the central nervous system. Intracerebroventricular injection of Ang II and Ang III produces equivalent pressor and drinking responses (58, 62). Ang III may be the main effector of vasopressin release in the brain (63). Further cleavage by aminopeptidase N converts Ang III to Ang IV, the hexapeptide Ang 3-8. The role of Ang IV on renal blood flow is somewhat controversial. Initial studies demonstrated that, in anesthetized rats, intrarenal infusion of Ang IV increased natriuresis and renal cortical blood flow, the effect of which was blocked by AT4 blockade, but not AT1 blockade (64, 65). Subsequent studies from multiple groups have shown that systemic and intrarenal Ang IV cause vasoconstriction and decrease renal blood flow, the effects of which are inhibited by AT1 antagonists, but not AT4 antagonists (66, 67, 68). Ang IV has also been implicated in the regulation of cell growth and the vascular inflammatory response in which it stimulates endothelial expression of PAI-1 (69). In vascular smooth muscle cells Ang IV activates the nuclear factor kB (NF-kB) pathway, leading to increased expression of monocyte chemoattractant protein-1 (MCP-1), intercellular adhesion molecule-1 (ICAM-1), interleukin-6, and TNF-α (70). Ang IV also plays a role in memory and cognition (71).
Angiotensin A (Ang A) and alamandine are two of the more novel peptides in the RAS system. Ang A is an octapeptide generated from the decarboxylation of the first residue of Ang II, Asp1, to Ala1 (72). Relative to Ang II, Ang A binds to AT1 with similar affinity and to AT2 with slightly greater affinity. As a result, the AT1-mediated effects of Ang A are not as potent as Ang II. Alamandine, which is formed from Ang A by ACE2 or through decarboxylation of Ang 1-7, has vasoactive effects similar to Ang 1-7 (73).
Angiotensins are also subject to hydrolysis by endopeptidases, the most likely of which to be physiologically relevant is neutral endopeptidase (NEP). NEP directly converts Ang I to Ang 1-7 (74). Ang 1-7 can also be formed from Ang II by prolyl endopeptidase and prolyl carboxypeptidase (75), as well as via cleavage by ACE2. Previously thought to be inactive, Ang 1-7 has been shown to induce renal afferent arteriolar vasodilatation (76) and to stimulate diuresis and natriuresis, possibly via inhibition of the Na+/K+ ATPase in the proximal tubule (77). In addition to its vasodilatory properties, Ang 1-7 decreases cardiac hypertrophy and fibrosis and prevents cardiac remodeling (78, 79). These actions appear to be mediated via binding to the G protein-coupled receptor Mas (79, 80). The ACE2-Ang(1, 2, 3, 4, 5, 6, 7)-Mas axis is thought to be a counterregulatory arm within the RAS, opposing the actions of Ang II (81).
Angiotensin Receptors
The actions of Ang II are mediated through binding to one of the Ang receptor subtypes. The two most well characterized are the type 1 and type 2 receptors, designated AT1 and AT2. Both receptor subtypes are G protein-coupled seven transmembrane receptors. Almost all known Ang II effects, including vasoconstriction, aldosterone secretion, increased sympathetic tone, and cellular growth and proliferation, are mediated by the AT1 receptor, although the functions of the AT2 receptor are increasingly being unraveled.
The AT1 receptor has been localized to multiple organs, including the brain, heart, adrenal gland, kidney, and vasculature (82). It is widely distributed throughout the heart, where Ang II binding causes positive inotropy and chronotropy, but receptor density is greatest within the conducting system (83). Ang II binding to AT1 receptors stimulates aldosterone secretion from the adrenal zona glomerulosa and catecholamine release from the chromaffin cells of the adrenal medulla. The high levels of the receptor throughout the vasculature on smooth muscle cells mediate changes in vascular tone due to Ang II. Within the kidney, AT1 receptors have been localized to the afferent arteriole, glomerular mesangial cells, renal medullary interstitial cells, vasa recta, and throughout the tubule (84, 85). Ang II stimulates sodium and water reabsorption, regulates the glomerular filtration rate (GFR), and inhibits renin secretion from the macula densa. Activation of AT1 receptors by Ang II stimulates cell growth and proliferation, including activation of the Janus kinases (JAK)/signal transducers and activators of transcription (STAT) pathway (86), expression of growth factors such as TGF-β1 and basic fibroblast growth factor (bFGF), and vascular smooth muscle cell and cardiac myocyte hypertrophy (87, 88, 89).
The AT2 receptor seems to oppose AT1 receptor-mediated effects. It is expressed in cardiac fibroblasts (90), the adrenal medulla (82), renal glomeruli, afferent arterioles, proximal tubule, and vasa recta (85, 91). Its abundance in kidney mesenchyme during fetal growth suggests an important role in normal development (92). However, AT2 receptor-knockout mice develop normally but have altered behavior and cardiovascular function, including an increased pressor response to Ang II infusion (93, 94). The AT2 receptor mediates production of vasodilatory substances in the kidney and induces natriuresis via a cascade involving bradykinin and nitric oxide (95). In contrast to the AT1 receptor, the AT2 receptor inhibits cell proliferation and promotes differentiation. Thus, a generally protective role for AT2 receptors has been suggested. For example, AT2 receptor-knockout mice suffer greater kidney injury than do wild-type mice in the partial renal ablation model of CKD (96).
Additional Ang receptors have been identified that are distinct from the AT1 and AT2 receptors. Ang IV binds with high affinity to the AT4 receptor but has poor affinity for the AT1 and AT2 subtypes. The AT4 receptor has been localized in multiple organs, including the kidney, heart, central nervous system, and adrenal gland (71). Insulin-regulated aminopeptidase (IRAP) has been proposed as the functional receptor for Ang IV (97), but this has been called into question (71). Data suggest Ang IV may exert its effects through c-Met, a type 1 tyrosine kinase inhibitor (98). The G protein-coupled receptor Mas has been identified as a functional receptor for Ang 1-7, as described earlier. Mas-knockout mice have impaired cardiac function and altered collagen expression toward a profibrotic state (99). The Mas-related G protein-coupled receptor D (MrgD) is the receptor for alamandine. Through the MrgD receptor, alamandine has been shown to have antihypertensive and antifibrotic effects in spontaneously hypertensive rats (SHR) (73).
Angiotensin II
Ang II is the principal effector of the RAS for the regulation of extracellular volume and blood pressure. It acts on multiple organs, including the heart, kidney, vascular system, adrenal gland, central nervous system, and intestine. The effects of Ang II on cardiovascular function include maintenance of systemic blood pressure via direct constriction of vascular smooth muscle cells, leading to increased systemic vascular resistance (SVR), and enhanced myocardial contractility. Ang II stimulates catecholamine release from the adrenal medulla and sympathetic nerve endings, increases sympathetic nervous system activity, and may enhance the vasoconstrictor response due to catecholamines (100, 101). Ang II acts to preserve extracellular volume via increased salt and water retention by stimulating aldosterone secretion from the adrenal glomerulosa, promoting thirst and water intake, and enhancing renal sodium transport.
In the kidney, Ang II directly affects renal hemodynamics, control of GFR, and tubular transport. The actions of Ang II in the kidney have been nicely reviewed by Ichikawa and Harris (102). In summary, Ang II causes arteriolar vasoconstriction, mediated primarily by protein kinase C (PKC) generation (103). It constricts both the afferent and efferent arterioles and the interlobular artery (104, 105, 106). Vascular resistance increases more
in the efferent than in the afferent arteriole in response to Ang II, partly due to the smaller resting diameter of the efferent arteriole (107). Thus renal blood flow declines and glomerular capillary hydraulic pressure rises, which preserves GFR in the setting of reduced systemic blood pressure.
in the efferent than in the afferent arteriole in response to Ang II, partly due to the smaller resting diameter of the efferent arteriole (107). Thus renal blood flow declines and glomerular capillary hydraulic pressure rises, which preserves GFR in the setting of reduced systemic blood pressure.
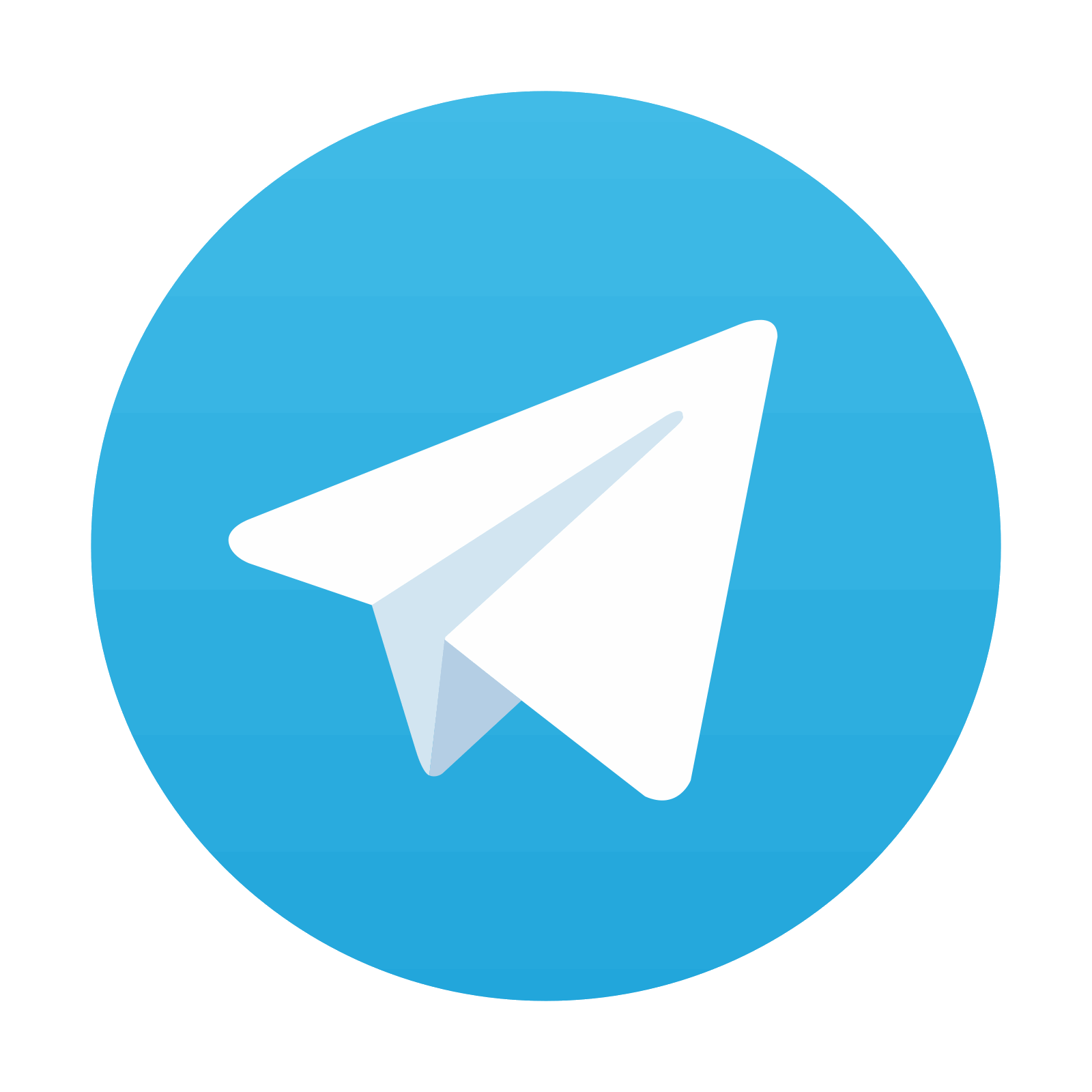
Stay updated, free articles. Join our Telegram channel
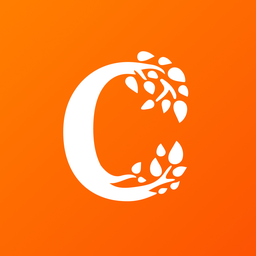
Full access? Get Clinical Tree
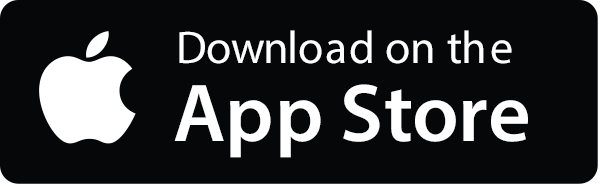
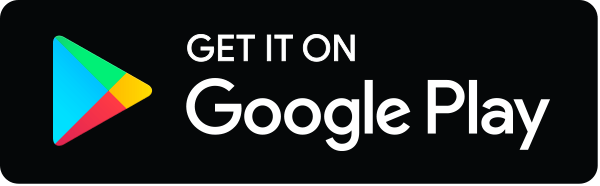