17 Janice Britt The term “pesticide” encompasses a group of chemical compounds that are used for the elimination or control of pests. Pesticides are grouped into classes based on their target of action. They comprise a diverse group as can be seen in Table 17.1. Table 17.1 Classes of Pesticides and Their Targets Pesticides have both economic and public health benefits. They are used for the control of vector-borne diseases (e.g., malaria, Lyme disease, West Nile Virus, avian flu, and Rocky Mountain spotted fever), for the promotion of agricultural production in the United States as well as in other countries, and by homeowners for the control of domestic pests (e.g., household and garden pests). More recently, agents such as vaporized hydrogen peroxide, methyl bromide, paraformaldehyde, and ethylene oxide have been approved as pesticides to be used against biological agents such as Bacillus anthracis (anthrax-producing bacteria that caused threats to national security in the United States). Individuals may be exposed to pesticides either occupationally (e.g., from working in a pesticide formulating plant or from commercial pesticide application) or environmentally (e.g., from food products such as fruits and vegetables treated for pests). Individuals may also be exposed to pesticides at their residences (e.g., from use as home or garden insecticide). The U.S. Environmental Protection Agency (USEPA) has recently reported the most commonly used pesticides (see Table 17.2). Table 17.2 Most Commonly Used Pesticides in the United States The registration and regulatory requirements concerning pesticides are governed under the Federal Insecticide, Fungicide, and Rodenticide Act (FIFRA). Rules aimed at the protection of agricultural and greenhouse workers who used pesticides were passed in 1992 by the USEPA and are located in 40 Code of Federal Regulations Parts 156 and 170. Occupational Safety and Health Administration (OSHA) as well as the American Conference of Governmental Industrial Hygienists (ACGIH) also publish guidelines for occupational exposures to pesticides in air. For some pesticides, the USEPA Integrated Risk Information System (IRIS) and/or the Office of Pesticide Programs (OPP) has set various guidelines for pesticides for drinking water (e.g., maximum contaminant levels and Human Health Benchmarks for Pesticides (HHBPs)) or in air (e.g., reference concentration (RfC)) as well as estimates of cancer risks (e.g., oral and/or inhalation slope factors). This chapter will discuss the classes of the most commonly used pesticides and will include a discussion of the following with respect to these pesticide classes: In the past, organophosphate compounds were used as replacements for the more persistent organochlorine insecticides because they did not bioaccumulate in tissues and organisms or accumulate in the environment. For example, chlorpyrifos, an organophosphate compound, was at one time, a widely used termiticide, serving as a substitute for the more persistent organochlorine compounds that were used in the past. However, several years ago, due to concern over toxicity of chlorpyrifos, the USEPA curtailed the use of this compound, with a few exceptions. Examples of organophosphate insecticides (and their active ingredient) include Dursban™ (chlorpyrifos), Knox Out™ (diazinon), and Vapona™ (dichlorvos) (see Figure 17.1 for examples of organophosphate insecticides). Examples of carbamate pesticide products (and their active ingredient) commonly used are Sevin™ (carbaryl) and Temik™ (aldicarb) (see Figure 17.2 for examples of carbamate insecticides). It should be noted that organophosphate compounds are not only used as pesticides; chemicals in this class are also used as therapeutic agents for the treatment of glaucoma and myasthenia gravis in humans. For example, the organophosphate echothiophate iodide is used to treat glaucoma. Rivastigmine (Exelon®), a reversible cholinesterase inhibitor, is currently being used to treat dementia. Currently, clinical investigators are exploring the possible use of other cholinesterase inhibitors to treat Alzheimer’s disease, Parkinson’s disease dementia, dementia with Lewy bodies, and other medical conditions. Figure 17.1 Examples of organophosphate insecticides. Figure 17.2 Examples of carbamate insecticides. Tetraethyl pyrophosphate (TEPP), the first anticholinesterase compound, was synthesized in the mid-1800s by the French chemist Philippe de Clermont and the Russian chemist Wladimir Moshnin who were students in Adolphe Wrutz’s laboratory in Paris, France. Schrader in Germany, who was developing toxic OP compounds during WWII, uncovered the insecticidal properties that TEPP possessed. While TEPP was an effective insecticidal compound, it was unstable in the environment, and so there was an effort to develop more stable compounds. This effort resulted in the development of organophosphate insecticides, including dimefox in 1940, schradan in 1942, and parathion in 1944. Both organophosphate and carbamate classes of compounds have the same mechanism of action in insects as well as in mammals (including humans)—the inhibition of the enzyme acetylcholinesterase. The inhibition of acetylcholinesterase by organophosphates and carbamates compounds is the mechanism of action that is responsible for the acute symptomatology associated with these compounds. Acetylcholinesterase is an enzyme located in the synaptic cleft, and its function is the breakdown of acetylcholine, the neurotransmitter present at the following sites: postganglionic parasympathetic nerves, somatic motor nerves endings in skeletal muscle, preganglionic fibers in the parasympathetic and sympathetic nerves, and in some synapses in the central nervous system. Organophosphate and carbamate insecticides act by inhibiting the enzyme acetylcholinesterase at its esteratic site, resulting in an accumulation of the neurotransmitter acetylcholine in nerve tissue and at the effector organ. This accumulation then results in the continued stimulation of cholinergic synapses and at sufficient level leads to the signs and symptoms associated with overexposure to these compounds (discussed later in this section). Organophosphates and carbamates can be readily absorbed via ingestion, dermal, and inhalation routes due to their lipophilic nature. Within the class of organophosphate insecticides, there are direct organophosphate inhibitors (those containing = O) and organophosphate indirect inhibitors (those containing = S), depending on whether or not they require metabolic activation before they can inhibit acetylcholinesterase. In other words, the indirect organophosphate compounds (containing = S) must undergo bioactivation to become biologically active (containing = O). The indirect inhibiting compounds, including organophosphates such as parathion, diazinon, malathion, and chlorpyrifos, become more toxic than the parent compound upon metabolism. In the case of these indirect inhibitors, oxidative desulfuration (replacement of the sulfur atom with an oxygen atom as described earlier) results in the formation of the oxon of the parent compound (e.g., parathion → paraoxon, diazinon → diazoxon, malathion → maloxon, and chlorpyrifos → chlorpyrifos-oxon). This metabolism occurs via the mixed function oxidase system of the liver. Organophosphate insecticides not requiring metabolic activation (i.e., direct inhibitors, discussed later) can produce local toxic effects at the site of exposure such as sweating (dermal exposure), miosis or pinpoint pupils (eye contact), and/or bronchospasms (inhalation exposure). Both the organophosphate and carbamate insecticides have relative short biological half-lives and are fairly rapidly metabolized and excreted. Once cholinesterase activity has been inhibited in the body by an organophosphate compound, the recovery of that compound is dependent on the reversal of inhibition, aging, and the rate of regeneration of a new enzyme. A chemical reaction that organophosphate insecticides can undergo in the body once they are bound to the cholinesterase enzyme is called “aging.” Aging involves the dealkylation of the compound once it is bound to the cholinesterase enzyme. In this “aged” form, the organophosphate compound is tightly bound to the enzyme and will not release itself from the enzyme. Once the aging reaction has occurred, treatment with medications (such as pralidoxime, discussed later) is not effective. Once the cholinesterase molecule has been irreversibly inhibited via the aging process, the only manner in which the enzyme activity may be restored is through synthesis of new enzyme. In addition to the aging reaction, organophosphates can undergo various phase I and II biotransformation pathways including oxidative, hydrolytic, GSH-mediated transfer and conjugation reactions. Carbamate compounds do not require metabolic activation in order to inhibit cholinesterase. Carbamate insecticides are not considered irreversible inhibitors like some organophosphate insecticides. Cholinesterase inhibition by carbamate compounds is readily reversible, with reversal of inhibition occurring typically within a few hours after exposure. This rapid reversal of the cholinesterase enzyme activity leads to a much shorter duration of action and thus a shorter period of intoxication than that seen in cases of organophosphate overexposure. Carbamates do not undergo “aging” as do the organophosphates. As with the organophosphates, carbamates also can undergo various phase I and II metabolism reactions. The effects of organophosphate and carbamate insecticides can be either local (e.g., sweating from localized dermal exposure as mentioned earlier) or systemic. Signs and symptoms of overexposure to organophosphate and carbamate compounds occur fairly rapidly after exposure, with effects typically seen beginning from 5 min to 12 h after exposure. A diagnosis of organophosphate intoxication typically is based on an exposure history of 6 h or less before the onset of signs and symptoms. It has been suggested that if symptoms appear more than 12 h after the exposure, then another etiology should be considered, and if the symptoms begin 24 h after the exposure, then organophosphate intoxication should be considered to be equivocal. Symptoms of carbamate overexposure generally develop within 15 min to 2 h of exposure and typically last only several hours, a duration much shorter than that of the typical overexposure to organophosphate pesticide. Symptoms that are present 24 h following exposure are likely not a result of overexposure to carbamate insecticides. The acute signs and symptoms seen in cases of overexposure to both organophosphate and carbamate pesticides are related to the degree of inhibition of acetylcholinesterase in the individual. The clinical manifestation of overexposure to organophosphate and carbamate compounds is a result of muscarinic, nicotinic and central nervous system symptoms (see Table 17.3). In systemic intoxications with organophosphate and carbamate compounds, the muscarinic effects are generally the first effects to develop. Table 17.3 Signs and Symptoms of OP Intoxication Organophosphate intoxication is diagnosed on clinical suspicion; an opportunity for exposure to the compound; odor of pesticides; signs and symptoms consistent with organophosphate overexposure; and significant inhibition (i.e., 50% inhibition) of cholinesterase enzyme as measured in the plasma and/or in red blood cells (this will be discussed later). Signs and symptoms resulting from overexposure to this organophosphate and carbamate compounds can be best described by the mnemonic DUMBELS: Diarrhea, Urination, Miosis (pinpoint pupils), Bronchospasm, Emesis (vomiting), Lacrimation (tearing), and Salivation. Signs and symptoms associated with exposure to organophosphates and carbamate compounds generally do not occur unless acetylcholinesterase activity is approximately 50% or less of normal activity. Signs and symptoms in cases of mild to moderate organophosphate intoxication typically resolve within days to weeks following exposure. In cases of severe organophosphate intoxication, it can be 3 months or so before cholinesterase red blood cell levels return to normal. Death from organophosphate intoxication is usually due to respiratory failure from depression of the respiratory center in the brain, paralysis of the respiratory muscles, and excessive bronchial secretions, pulmonary edema, and bronchoconstriction. Death in individuals with acute organophosphate intoxication that are untreated typically occur within the first 24 h, and within 10 days in treated individuals. If there is no anoxia, complete recovery will occur, in general, within about 10 days after the exposure incident. Carbamate intoxication presents similar to that of organophosphate intoxication. Cases of carbamate intoxication resolve much more quickly than cases of organophosphate overexposure due to the rapid reversal of acetylcholinesterase enzyme with the carbamate insecticides as well as to the rapid biotransformation in vivo. In general, the main reported chronic effect that may result from exposure to organophosphate insecticides is delayed neuropathy. Organophosphate-induced delayed neuropathy (OPIDN) has been associated with exposure to only a few organophosphate compounds, with cases of OPIDN having occurred almost exclusively at near-lethal exposure levels. No permanent effects generally result from carbamate intoxication; delayed neuropathy does not occur as a result of carbamate poisoning (see discussion later). A few of the organophosphates have been associated with the development of a rare condition—a delayed predominantly motor peripheral neuropathy, termed OPIDN. In the United States in the 1930s, individuals developed OPIDN, also called “Ginger Jake” paralysis, after consuming ginger liquor contaminated with triorthyl cresyl phosphate (TOCP). Other outbreaks of OPIDN have occurred in relation to the consumption of cooking oil contaminated with TOCP. Organophosphates that have been associated with OPIDN include TOCP, mipafox, trichlorphon, leptophos, and methamidophos. It should be pointed out that only a few of the organophosphate compounds actually are capable of causing OPIDN. The development of OPIDN is not physiologically related to cholinesterase inhibition. The nerve lesion in OPIDN is that of a distal symmetric predominantly motor polyneuropathy of the long, large diameter axons (the short, small diameter nerves appear to be spared) in the peripheral nerves. OPIDN, as its name suggests, has a delayed onset of approximately 1–3 weeks following an acute life-threatening exposure to an organophosphate capable of causing delayed neuropathy. The initial complaints of OPIDN include cramping of the calves with numbness and tingling in the feet and sometimes in the hands in severe cases. Next, weakness develops in the lower limbs. Bilateral foot drop and wrist drop may develop, and there are usually absent or normal reflexes. A high-stepping gait has also been described in individuals with OPIDN. There also may be motor weakness involving the limbs and motor nerve conduction studies may show abnormalities. The current theory as to the cause of OPIDN is a two-step process that occurs in the nervous system. First, it is thought that phosphorylation of a target protein in the nervous system is required. This enzyme is known as neuropathy target esterase (NTE), formerly known as neurotoxic esterase. The biological action of NTE in the body is not known. The second, and essential, step leading to OPIDN is thought to be the transformation, or “aging” of the enzyme. This “aging” process involves cleavage of a R-group from phosphorous, resulting in a negatively charged residue attached to the active site of the enzyme. It appears that compounds that are capable of inhibiting NTE and aging can only cause OPIDN if a threshold of inhibition is reached. A high level of inhibition—70 to 80% inhibition of NTE in the brain, spinal cord, or peripheral nerve of the experimental animal—soon after dosing with an organophosphate capable of causing OPIDN is necessary before this condition can develop. Thus, the determining factor in the development of OPIDN is the formation of a critical mass of aged-inhibited NTE. A term used to express the concentration of a substrate (such as an organophosphate compound) that is needed to inhibit 50% of an enzyme is known as the IC50. One way to predict whether or not a compound will produce OPIDN is to compare AChE and NTE IC50s for a specific compound. The in vitro and in vivo IC50s for NTE and AChE in humans and hens (the test species used to evaluate the delayed neuropathic potential for organophosphate insecticides) for several organophosphate compounds have been compared, and it was found that for organophosphate compounds with a IC50 AChE/IC50 NTE ratio of less than one, OPIDN can only occur after recovery and treatment from acute, otherwise fatal, cholinergic crisis. The scientific literature indicates that for at least the few compounds known to cause OPIDN that have been analyzed, the AChE IC50/NTE IC50 typically is less than unity. This means that the concentration of a chemical that will inhibit 50% of the AChE molecules is less than the concentration of the same chemical that is required to inhibit 50% of the NTE molecules. Therefore, at a given concentration, AChE will be inhibited to a greater degree than NTE. As previously stated, it is currently theorized that more than 50% of NTE (i.e., 70–80%) must be inhibited in order to develop OPIDN. Likewise, a 50–80% inhibition of AChE results in clinical manifestations. In fact, human case reports indicate that virtually all patients who develop OPIDN were managed for a cholinergic crisis first. It important to note that carbamates do not caused delayed neuropathy. While some carbamates are capable of inhibiting NTE, aging does not occur. In fact, experimental evidence has showed that some carbamates that inhibit NTE actually protect hens against developing OPIDN. The organophosphates and carbamates have the ability to inhibit pseudocholinesterase, red blood cell cholinesterase, and nervous system cholinesterase, with biological effects being due to the actual inhibition of nervous system cholinesterase only. The levels of cholinesterase present in the blood, especially in the red blood cells, can be used to estimate the degree the nervous system is being affected by anticholinesterases. As mentioned earlier, a 50% depression of plasma and red blood cell cholinesterase levels is typically necessary before clinical manifestations are seen. Acute overexposures to organophosphates can be classified as mild (20–50% of baseline cholinesterase levels), moderate (10–20% of baseline cholinesterase levels), or severe (10% or less of baseline cholinesterase level). Plasma cholinesterase, while susceptible to the inhibitory actions of organophosphate insecticides, has no known biological use in the body. Plasma cholinesterase can vary in an individual based on a number of disease states or conditions (e.g., decreased plasma cholinesterase levels in liver disease such as cirrhosis and hepatitis, multiple metastases, during pregnancy). Plasma cholinesterase is produced by the liver, and this enzyme is found in the nervous tissue, heart, pancreas, and in white matter of the brain. Plasma cholinesterase levels typically decline and regenerate more rapidly than red blood cell cholinesterase levels. Plasma cholinesterase levels typically regenerate at the rate of 25% in the first 7–10 days. Following organophosphate intoxication, plasma cholinesterase levels may remain depressed for a period of 1–3 weeks. The enzyme in red blood cell cholinesterase is the same enzyme that is present in the nervous system. Red blood cell cholinesterase regenerates at the rate of approximately 1% per day in the body and is dependent on the synthesis of new red blood cells in the body. As mentioned earlier, in severe intoxications from organophosphate pesticide exposure, red blood cell cholinesterase could take as long as 3 months to regenerate. The measurement of cholinesterase activity in cases of carbamate intoxication is not useful due to quick reactivation of cholinesterase following carbamate overexposures. Each year, approximately 3 million individuals suffer from OP intoxication worldwide, resulting in about 300,000 deaths. Historically, two treatments have been used in cases of organophosphate intoxication: (i) atropine (muscarinic antagonist) and (ii) an oxime medication such as pralidoxime or 2-PAM (reactivates acetylcholinesterase). Atropine competes with muscarinic sites, and treatment with the agent ameliorates the symptoms of nausea, vomiting, abdominal cramps, sweating, salivation, and miosis. Atropine treatment has no effect on the nicotinic signs, such as muscle fasciculations, muscle weakness, or respiratory failure. Atropine does not reactivate cholinesterase.
PROPERTIES AND EFFECTS OF PESTICIDES
Class
Target
Acaricides
Mites and ticks
Algicide
Algae
Antifouling agents
Organisms that attach to underwater surfaces
Antimicrobials
Bacteria and viruses
Attractant
Attracts pests
Avicides
Birds
Disinfectants/sanitizers
Kills or inactivates microorganisms on inanimate objects
Fumigants
Pests in buildings or soil
Fungicides
Fungi
Herbicides
Weeds or undesirable plants
Insecticides
Insects
Larvicides
Larvae such as mosquitoes
Miticides
Mites
Molluscicides
Slugs and snails
Nematicides
Nematodes
Ovicides
Eggs of pests (e.g., fleas)
Piscicide
Fish
Repellants
Repels pests such as mosquitoes
Rodenticides
Rats, mice, and other rodents
Silvicide
Trees, shrubs, or forests
Termiticide
Termites and ants
Most Commonly Used Pesticides in U.S. Agricultural Market Sector
Glyphosate
Atrazine
Metam sodium
Metolachlor-S
Acetochlor
Most Commonly Used Pesticides in Non-Agricultural Sectors of the United States
Home and Garden Market
2,4-Dichlorophenoxyacetic acid
Glyphosate
Carbaryl
MCPP
Pendimethalin
Industrial/commercial/government uses
2,4-Dichlorophenoxyacetic acid
Glyphosate
Chlorothalonil
MSMA
Diuron
17.1 Organophosphate and Carbamate Insecticides
Introduction, Use, and History of Organophosphates and Carbamates
Mechanism of Action
Absorption and Metabolism
Acute Effects of Organophosphate and Carbamate Insecticides
Muscarinic (Parasympathetic Nervous System)
Nicotinic (Sympathetic and Motor Nervous Systems)
Nicotinic and Muscarinic (Central Nervous System)
Increased salivation
Sweating
Giddiness
Increase lacrimation
Pallor
Tension
Bronchospasm
Hypertension
Anxiety
Restless
Muscle fasciculations
Restlessness
Dyspnea
Muscle cramps
Insomnia
Anorexia
Motor weakness
Nightmares
Nausea
Tachycardia (↑ heart rate)
Headache
Vomiting
Paralysis
Tremors
Abdominal cramps
Drowsiness
Diarrhea
Confusions
Miosis (pinpoint pupils)
Slurred speech
Blurred vision
Ataxia
Urinary frequency and incontinence
Cheyne–Stokes respiration
Bradycardia (↓ heart rate)
Convulsions
Hypotension
Coma
Cyanosis
Chronic Effects of Organophosphate and Carbamate Insecticides
Organophosphate-Induced Delayed Neuropathy
Biological Monitoring for Organophosphates and Carbamates
Treatment for Organophosphate and Carbamate Intoxication Symptomatology
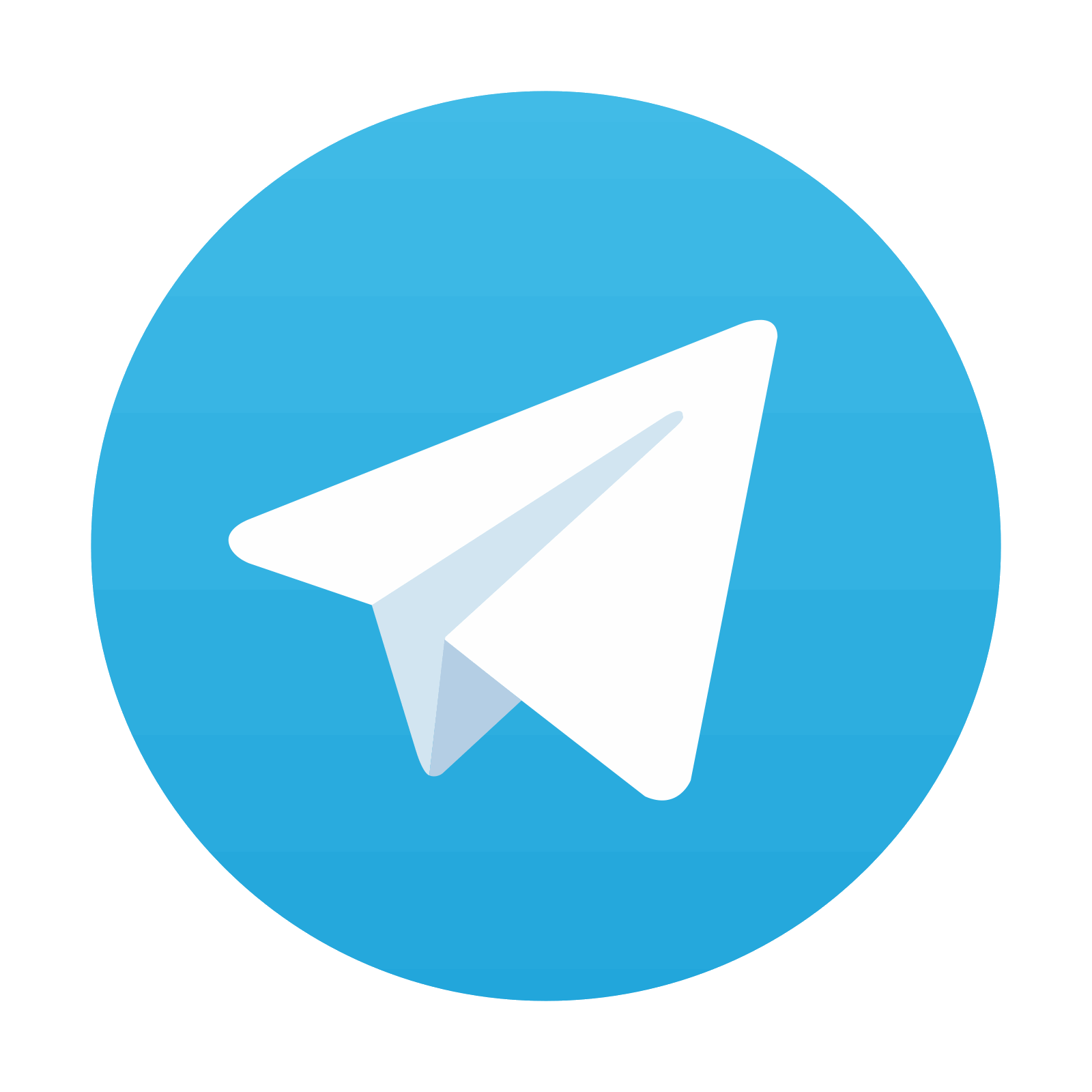
Stay updated, free articles. Join our Telegram channel
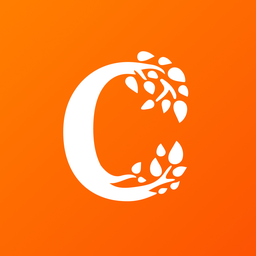
Full access? Get Clinical Tree
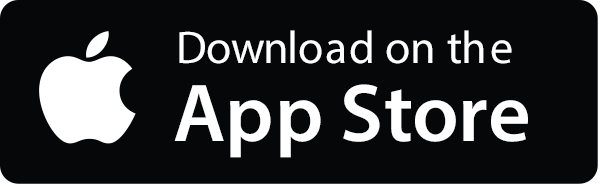
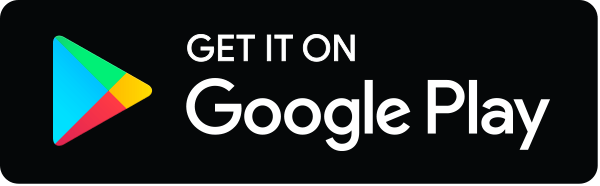