Fig. 8.1
Oxyhemoglobin dissociation curve
Conventional Wisdom Can Be Harmful
The Oxyhemoglobin Dissociation Curve (ODC), discovered in 1903 by Christian Bohr, was changed substantially in the mid 1980s because of our scientific infatuation with reductionism and the over simplified methodology of Threshold Science, also helped along with an ‘educational interest’ surge in our society to ‘go metric’ [6]. Because of these influences, the so called ‘knee’ of the curve was conceptualized and disseminated with the introduction of pulse oximetry into our OR, PACU, and Critical Care environments. Those responsible for propagating the concept, namely the oximetry industry, had really not thought this through very well, as we shall explain.
The gist of the ODC ‘knee’ construct was that medicine was indeed fortunate to have a precise oximetric SPO2 (blood oxygen saturation) threshold value that could reliably determine whether or not respiratory function was stable and well. So long as we maintain oxygen saturations at 90 % and above, all is well, but let it slip just a bit below 90 % and watch out! … nothing short of a free fall spiral of respiratory dysfunction that must be corrected immediately to avoid grave consequences. This ‘knee’ threshold, depicted at the green oval that sits on the curve’s 90 % SPO2 mark in Fig. 8.1, and everything leftward of this curve’s mark indicates the sharp, potentially lethal drop-off advertised. While it is difficult to quantify how much damage a misleading belief like this may have caused, here are two explanations for why this belief should be abandoned.
Reasons to Dispel Oxyhemoglobin Dissociation Curve ‘Knee’ Construct
Evolutionary Disconnect
We all have inherited any number of astonishingly efficient compensatory mechanisms, summoned automatically with injury. We’ve known for a century that an amazing amount of resiliency is packed into all our organ systems, including:
The ability to increase our oxygen supply 9 fold through coordinated increases in our heart rates, cardiac performance, and ventilatory responses
Remodeling once organs have been damaged
Regeneration, as our livers are able to accomplish under certain circumstances
So why might our moment to moment management of oxygen, the most primal of human needs, be so fragile that it could outright fail simply by breaching a metrically friendly (100–10 %) threshold SPO2 value located at the ODC ‘knee’? The correct answer – it’s not! We just momentarily got our interpretation of the curve turned backward. Instead of interpreting the curve’s steep dive from its knee as being a problem, we should appreciate its immediately narrowed range of O2 partial pressures (x axis) preserved during this accelerating saturation decline (The magic lives here in Fig. 8.1). It’s these partial pressures that are responsible for transferring oxygen molecules across the multiple bio-barriers and ultimately into our mitochondria for aerobic processing. In order for this to happen, we need a 42 mmHg PcO2 pressure head in our capillary beds to ultimately penetrate our intracellular mitochondria and their 26 mmHg PMO2 steady states. Seen this way, any falloff from the knee automatically deploys an evolutionary marvel designed to preserve aerobic metabolism at all costs. It’s our last wall of defense, protecting us from shock like states that otherwise would arise from even the most insignificant, transient episodes of hypoxemia.
Naïve Interpretation of Respiratory Stability
SpO2 values well above 90 % on continuous pulse oximeters can be deceiving and should never be considered sentinels of respiratory contentment when patients complain they are short of breath. Any patient can maintain a ‘good’ saturation early while slipping toward the RECC clinical abyss because of compensatory hyperventilation. The patient’s actual unfavorably declining PaO2 changes remain concealed because of this compensatory adaptation. Respiratory alkalosis from hyperventilation and its effect on hemoglobin affinity hold saturations stable almost immediately in early crisis. Our compensatory actions will deliver oxygen first and foremost when the respiratory system is challenged. Only later in the process does hydrogen ion stability, conformational changes of the hemoglobin molecule, and ultimately a precipitous fall in oxygen saturation (SpO2) combine to produce a resounding state of total respiratory collapse. Unfortunately this is when we most often are just beginning to realize our patients really are in trouble, when it’s difficult to miss and much more difficult to reverse, with death likely to follow quickly. We will explore the wonders of the Oxyhemoglobin Dissociation Curve (ODC) more thoroughly with our in depth review of RECC Type II PUHD.
Designing the Solution
Now we’re ready to begin explaining our three patterns of unexpected hospital death associated with RECC. These patterns don’t account for all the ways people die in hospitals, but they do define those rapidly progressing, unexpected clinical processes persistently taking us by surprise. They’re routinely associated with catastrophic outcomes because of delayed detection, and their prevalence hasn’t changed in two decades regardless the ‘advances’ in monitoring technologies. Don’t be overly concerned if you don’t immediately grasp the context of our three constructs. All will become clear more quickly than you think.
The Three RECC Pattern Types of Unexpected Hospital Death
TYPE I: Hyperventilation Compensated Respiratory Distress (e.g. Sepsis, Congestive Heart Failure, Aspiration, Pulmonary Embolism). Here a stable SpO2 (blood oxygen saturation) with progressively falling PaCO2 (arterial partial pressure of carbon dioxide) eventually yields to a slow SpO2 decline (mitigated by compensatory respiratory alkalosis shifting the oxyhemoglobin dissociation curve to the left), then followed by a precipitous SpO2 decline when metabolic acidosis dominates nearing its terminal stages.
TYPE II: Progressive Unidirectional Hypoventilation (CO 2 Narcosis, overdose of opioids or sedatives). Here one finds a progressive rise rather than the usual rise and leveling off of an elevated PaCO2 and etCO2 (end tidal carbon dioxide), and a concurrent fall in SpO2 over 15 min to many hours once the PACO2 (alveolar partial pressure of CO2) becomes high enough to begin competing with the PAO2 (alveolar partial pressure of oxygen) for alveolar space.
TYPE III: Sentinel Rapid Airflow/SpO 2 Reductions Followed by Precipitous Falls in SpO 2 (A state of ‘arousal dependent survival’ that occurs only during sleep in sleep disordered breathing conditions such as obstructive sleep apnea and some hypoventilation syndromes). Here one finds a preconditioned degree of arousal failure often partially enabled by a decrease in oxygen reserves that allows for a precipitous hypoxemia event during apnea causing a terminal arousal arrest.
Linking the Patterns to Physiologic Principles (Understanding Lung Capacities and Volumes – Ventilatory Anatomy and Physiology)
Before we begin our deeper looks into each of our three RECC PUHD Types, we’ll take a moment to review some basic pulmonary science. This will provide us an easy way to both understand and remember the unique patho-physiologic processes that define each PUHD.
The diagram in Fig. 8.2 illustrates what we mean when we discuss lung capacities and volumes.


Fig. 8.2
Lung capacities and volumes
Functional Residual Capacity (FRC) depicted above in Fig. 8.2 is an important, albeit virtual anatomic structure whose job is to continually provide our bodies any additionally needed oxygen beyond that being delivered within our moment to moment tidal volumes, so to maintain the stability our arterial oxygen content. It functions largely as an oxygen reservoir, playing a vitally important role as a necessary and persistent contributor to our respiratory physiology, maintaining our generally very stable arterial oxygen saturations. FRC is a combination of two real and separate anatomic volumes called Expiratory Reserve Volume and Residual Volume, but is more easily remembered as the lung air left over after normal exhalation. Our lungs hold approximately 6 L of air for men and less than 5 L for women at full capacity, achieved only during deepest inspiration. But on average our lungs normally operate at rest with our taking in tidal volume (VT) breaths of 500 ml ‘atop’ our FRC. Exhalation occurs approximately 16 times a minute leaving on average 2 L of air behind. Without our FRC, the tidal volumes we depend on to ‘freshen’ our FRC would only be capable of introducing oxygen into our circulations during a small portion of our ventilatory cycles. The FRC adds a comfortable cushion, allowing for continual restocking of oxygen desaturated blood that recurs reliably (to a point) even when lungs are partially damaged or breathing stops over short time intervals, like when holding our breaths, or at the onset of being strangled. Unfortunately, more prolonged apneas can deplete this FRC reservoir regardless how robust it might be under normal circumstances, which is germane to our coming discussion on Obstructive Sleep Apnea and RECC Type III PUHD. But our primary reason for discussing FRC is that all three RECC pattern types can be easily remembered by associating each with a purposefully exaggerated, distinctly separate pathologic influence it inflicts on the FRC.
These three exaggerated pathologic FRC influences are defined with one word each, except for the last (RECC Type III), requiring two words. Exaggerations were purposefully chosen because they have proven to be the most reliable memory cues available. Recall association always works best when using unusual or absurd cues. These memory cues enable bedside clinicians to quickly, easily, and reliably recall how to approach GCF patients regarding each of our three RECC Types. Critical thinking, what constitutes an appropriate response, what doesn’t and why, all of this remains fresh and available to any clinician using these cues. The importance of ongoing monitoring and paying attention to trends becomes obvious and engaging as well, even though the cues aren’t meant to be precise. Respiratory dysfunction that involves acute progressive hypoxemia is a complex subject, comprising a myriad of supply and demand contributors that include ventilation/perfusion mismatching, shifting closing pressures, hypoventilation, apnea, FRC reductions, atelectasis, diffusion failure, shunting, and low venous saturations to name just a few. But our simplified FRC associated cues make this complexity vanish and appropriate early correction possible, the RECC Types I, II, III now recognizable early and understood. This should translate to providing patients their best chances to avoid needless complications, or if unavoidable, to limit morbidity. And now we shall begin our deep looks into our RECC PUHD Types.
RECC Type I PUHD
A healthy male who had just undergone elective surgery develops shortness of breath that’s noticed by his family who express concern to the nurse. The nurse, citing a normal oxygen saturation reading on his oximeter, reassures the family that the monitor indicates he’s okay. Eventually his respiratory rate does rise to a critical value, but by this time it’s too late to effectively respond to his rapidly deteriorating clinical condition and the patient, with sepsis, dies.
This pattern, defined as Hyperventilation Compensated Respiratory Distress, reflects a clinically evolving process associated with microcirculatory failure induced by such common conditions as sepsis, congestive heart failure (CHF), aspiration, and pulmonary embolism (PE). It’s the most common pattern of our three, with incidences reaching as high as 3 % in some postoperative populations. Let’s first examine how the onsets of our four RECC examples of Type I PUHD might compromise the FRC and its ability to stabilize oxygen saturation. The word cue to describe this Type I process is ‘replacement’. RECC Type I PUHD involve processes that replace a patient’s healthy lung (FRC) acutely and near immediately. With CHF, water does this replacing. In sepsis, it’s pus (inflammatory factors). It’s gastric and bowel content with eventual pus in cases of aspiration, and with PE, portions of the FRC vanish outright to become dead space. Targeting the correct replacement process early, before a critical mass of lung becomes irreversibly injured, is essential to survival.
Type I patterns generally begin with subtle hyperventilation and a persisting respiratory alkalosis (RA), despite subsequent progressive increases in anion gap and lactic acid levels. This stage occurs well before the development of dominant metabolic acidosis (MA), which is usually associated with its later, and very late terminal stages. These progressive pattern phases (initially isolated RA followed by mixed RA and MA, in turn followed by dominant MA) comprise the typical progression of a Type I PUHD. (Illustrated in Fig. 8.3 below.)


Fig. 8.3
RECC Type I PUHD
The subtle early signs are easily overlooked, usually accompanied with complaints of mild dyspnea (shortness of breath) if patients are able to articulate their symptoms, and often mistaken for anxiety. Nurses and physicians have been too willing to discount these harbingers, even more so today than back in the pre-oximetry era, because now with oximetry often available patients may begin complaining, but their SpO2 values are seen as remaining ‘normal’. Saturations in the mid-90 % are commonly misinterpreted as indicating respiratory stability when it’s really not. What’s forgotten is that the subtle hyperventilation accompanying early complaints of dyspnea can easily mask the troubling changes taking place in the patient’s blood and lungs by shifting the Oxyhemoglobin Dissociation Curve (ODC) leftward. Here time becomes the patient’s most important resource…using it appropriately can be life-saving! FRC replacement is actually a progressive injury developing in the lungs where oxygen saturation at first holds steady from the compensatory respiratory alkalosis while PaO2 begins to diminish. Caregivers walk away falsely reassured because they’ve forgotten their basic science. Any complaints or signs of dyspnea need to always be carefully evaluated at their onset! Unfortunately, this frequently isn’t the case. Instead, very high respiratory rates (≥30/min) eventually trigger rapid response team activations in most hospitals [16, 17], likewise triggering these patients’ first detailed evaluations, both late and sadly most often found in the non survivors when examined retrospectively [18].
Mildly elevated respiratory rates are known to be nonspecific markers for respiratory distress, and are often ignored or unreliably recorded until extreme values are reached. Once reached, like high lactate levels [19], they become specific, but for the much later RECC Type I PUHD manifestations of severe metabolic acidosis. Here they are best considered markers of severity and diagnostic delay [20] rather than useful warnings of early instability. Late Type I PUHD is both sensitive and specific for an irreversible condition, and both the misinterpretation of pulse oximetry and the unreliable recordings of respiratory rate have combined to contribute to, rather than counter Type I events. However, now available is an accurate, continuous non-invasive minute ventilation monitor that could be combined with pulse oximetry to provide the most reliable information needed for early detection of all Type I PUHD events, and Type II and III as well…more to come on this subject later.
Once again, early microcirculatory failure in the lungs begins a progressive fall in PaO2 and available oxygen content [21] as you might imagine the patient’s FRC starting to be replaced. But the accompanying patient hyperventilation perpetuates normal appearing oxygen saturation (SpO2) values because of its associated respiratory alkalosis and the ODC’s leftward shift [22]. These early compensatory changes can fool uninformed clinicians with initial, ‘normal’ appearing saturations that disguise this RECC. By thinking it through, early detection and intervention is possible. But what more commonly follows makes matters worse. Supplemental oxygen is ordered, starting at ‘low’ levels, then increased in increments, where it continues to conceal the advancing pathologic replacement changes, all the while the patient’s condition deteriorating.
The iterative increases in supplemental oxygen mask and delay accurate assessment by matching the dynamically advancing replacement (injury) process with its own dynamic concealment process. The oximeter and its SpO2 values remain falsely reassuring until quite late when the RECC Type I PUHD turns deadly. These routine delays in detection and management are commonplace on our general care floors. When reviewed, these events show both physicians and nurses lulled into early complacency. Then hours go by with the only documented ‘management’ being incremental increases in the supplemental oxygen that perpetuates this complacency. These passive tactics, rather than workups and aggressive treatments these situations call for, become causative! They are directly associated with the inevitable dismal outcomes, but rarely appreciated as culpable. Patients generally get whisked off to Critical Care Units, considered only victims of expected perioperative risk. And the cavalier misuse of supplemental oxygen continues, mistakenly condoned or ignored from ignorance. Death, when it does come this way, is rarely regarded catastrophic except within those families involved … though it ought to be. Remember, supplemental oxygen should only be used in tandem with an aggressive search for a possible underlying RECC process. Otherwise, it unfortunately becomes the second way supplemental oxygen can harm.
RECC Type II PUHD
A healthy female who is receiving routine post–op nasal oxygen has been up all night complaining of severe post-op pain, but is now finally asleep after yet another dose of IV opioid. The nurse, noticing on rounds the patient’s oxygen saturation is ‘perfect’ on the monitor, decides not to awaken her. She is found dead in bed 4 h later.
This pattern is best defined as Progressive Unidirectional Hypoventilation (CO2 Narcosis). Since the 1950s [23], nurses and physicians in training have learned that opioids produce death through a singular path involving progressive hypoventilation. The combination of opioids and a rising PaCO2 contributes to central depression of our ventilatory drive, ultimately leading to ‘CO2 Narcosis’ severe enough to bring on respiratory arrest. Opioid associated events aren’t unusual in hospitals today. Experts speculate that up to a third of all code blue arrests in hospitals could result from opioid induced respiratory depression [24], and naloxone is administered as an antidote for opioid associated events in 0.2–0.7 of patients receiving them postoperatively [25, 26]. One estimate has these representing 20,000 of our nation’s patients annually with one tenth suffering significant opioid related injuries that include death [27]. When severe injury and death do result from opioids lawfully administered in hospitals, it’s termed ‘iatrogenic’, arguably a less pejorative way to say we clinicians are responsible. These events are always catastrophic, devastating to patients, patient families, and all clinicians involved. Yet in spite of all this incentive to improve, we have not made meaningful progress in protecting our patients from these events for a host of reasons, one being the increased emphasis on optimal postoperative pain management by centers that govern reimbursement [3].
The RECC Type II Pattern exhibits a significant diminution in both inspiration and expiration airflow that results in significant quantitative changes in the amounts of gases normally occupying lung. Because these gas partial pressure shifts equilibrate near immediately with arterial blood minus a small, generally predictable A-a gradient, once extreme levels are reached, they can cause biochemical dysfunction at all end organ sites, most importantly the brain. Specifically, PaCO2 increases within the blood and PaCO2 in lung, unable to be cleared because of the opioid induced ventilatory depression. When levels of PaCO2 have climbed to ‘narcotizing’ thresholds in the 70 mmHg range, carbon dioxide’s own respiratory depressive effect begins to assert, combining synergistically with the opioid to accelerate a covert respiratory failure, and with it both morbid obtundation and severe respiratory acidosis. On the postoperative GCF, these progressing respiratory failures are often confused with blissful sleep while extraordinarily high PaCO2 levels continue to silently mount. These Type II events are frequently discovered only by accident when unsuccessful attempts are made to arouse these patients. They’re the lucky ones!
Some patients are at very high risk for postoperative hypoventilation when given ‘normal appearing’ doses of sedatives and opioids. Patients with congenital central hypoventilation syndrome [28] can be completely asymptomatic while awake, yet despite their normal daytime PaCO2, exhibit profound hypoventilation responses to sedation and opioids when asleep. Others at risk include patients with obesity hypoventilation syndrome [29], chest wall deformities, polio sequelae, advanced COPD [30], and severe hypothyroidism [31].
Our current practice for detecting opioid induced respiratory depression is to monitor the respiratory rate, and while some studies have shown that respiratory rate reductions provide a useful indication of ventilatory depression in some patients [32, 33], there’s ample evidence to suggest that it’s not quite that simple. Several studies have shown narcotic and sedative induced respiratory depression frequently associated with reductions in tidal volume and more variable patterns of breathing [34–36]. In fact, hypoventilation produced by some benzodiazepines may primarily reduce tidal volumes with accompanying increases in respiratory rate [37]. In obese patients, or others with narrow, semi-patulous upper airways, tidal volumes may be even further reduced through increases in opioid associated upper airway resistance [7, 8], suggesting that any relative reductions in rate and/or tidal volume are likely to be highly variable depending on both patient and drug-related factors. Because, in so many cases, tidal volume may be reduced to a significantly greater extent than respiratory rate, the application of threshold respiratory rate monitoring as our single surrogate marker for opioid induced respiratory depression can easily provide a false sense of security. The addition of continuous pulse oximetry surveillance to intermittent or continuous respiratory rate monitoring can be just as inadequate when supplemental oxygen is being provided [38].
As hypoventilation progresses, if supplemental oxygen is being provided, it can disguise the CO2 retention and progressively rising PaCO2 from continuous pulse oximetry monitoring until very late when lethal levels have accumulated [39–41]. This masking effect can result in deadly delays much like it does with Type I PUHD, but for an entirely different reason. Without supplemental O2, the expected Fraction of Inspired Oxygen (FIO2) contained in the air we entrain into our lungs is more quickly diluted into much leaner partial pressures through a FRC ‘substitution’ process. Where Type I PUHD can be thought of as ‘replacing’ the FRC itself, Type II PUHD ‘substitutes’ one gas’s progressively rising partial pressures (PACO2 and PaCO2) for the partial pressures of those others remaining from the inhaled room air (N2, H2O, and O2), a biologic dilution that when added all together equals the expected atmospheric pressure. This trapped, progressively rising PACO2 competes for the same ‘FRC’ space as the fixed partial pressure components of air, progressively diminishing the latter, most importantly P AO2. The alveolar gas equation:
can approximate what these expected partial pressure values of the diluted (substituted) alveolar oxygen should be in people with lungs that function normally. For example, at sea level breathing room air with a normal PaCO2 of 40 mmHg, we can expect the PAO2 to be 99.7 mmHg. If the PaCO2 rises to 50 mmHg, the PAO2 falls to 87.2 mmHg, 60 mmHg PaCO2 = 74.7 mmHg PAO2, and 70 mmHg PaCO2 (CO2 Narcosis) = 62.2 mmHg PAO2. Assuming normal breathing patterns and pulmonary physiology, it isn’t difficult to reasonably estimate from the PAO2 what the PaO2 should be. Generally, the PAO2-PaO2 (A-a gradient) when breathing room air can be calculated simply by taking a patient’s age, adding 10 to this value, and dividing the sum by 4. So if a 50 year old patient breathing room air has a PAO2 rounded off to 100 mmHg, his PaO2 would be expected to approximate 85 mmHg (15 mmHg A-a gradient). And once we know the PaO2, we should be able to estimate his blood oxygen saturation (SPO2) with reasonable accuracy, but only if we account for the variables that influence hemoglobin saturation. These variables are the parameters capable of shifting the Oxyhemoglobin Dissociation Curve (ODC) to the right or left, such as concurrent arterial blood pH, PaCO2, background 2,3DPG (Diphosphoglycerate), and temperature. There may be hidden variables as well, unique to individual patients that can skew any of these mathematical outcomes unexpectedly. However, generally automated mathematical models like HbO.Severinghaus and HbO.Dash are used today to correlate reliable saturation values off any known PaO2, provided we accept certain assumed fixed values for some of the less important variables, yet account for the most important ones. The HbO.Dash model offers one large correction advantage by allowing us to factor the patient’s concurrent arterial blood pH and PaCO2 into its saturation determination. This provides a significant correction because it accounts for the Bohr effect, a major physiologic modifier that shifts the ODC increasingly rightward or left, the more acidotic or alkalotic a patient’s arterial blood might be. Respiratory acidosis (elevated PaCO2) and its rightward curve shifts can significantly decrease the expected saturation values on any given PaO2. Leftward shifts associated with respiratory alkalosis (diminished PaCO2) raise expected saturation values for any given PaO2, like that caused by the hyperventilatory respiratory alkalosis seen in early Type I PUHD. The same ODC shifts holds true for metabolic acidosis and alkalosis.
![$$ \left[{P}_A{O}_2={F}_I{O}_2\left({P}_{A TM}- P{H}_2 O\right)- P aC{O}_2/ RQ\right] $$](https://i0.wp.com/basicmedicalkey.com/wp-content/uploads/2017/08/A304849_1_En_8_Chapter_Equa.gif?w=960)
Regarding our RECC Type II PUHD, an immediate mounting respiratory acidosis can be assumed from its definition of rapidly evolving unidirectional hypoventilation that results in progressive CO2 retention. With immediate onsets of respiratory acidosis, an arterial blood’s pH value in an otherwise healthy individual can be predicted directly from knowing the PaCO2 value using a Henderson-Hasselbach equation calculator (pH = 6.1 + log(HCO3/(0.03 × PaCO2)), because the equation’s bicarbonate (HCO3) variable remains fixed at 24 mEq/L. (It generally takes 3–5 days for our kidneys to begin compensating for any acute respiratory acidosis by preserving additional HCO3) Pulse oximeters measure saturation directly and don’t sort out which variables are at work altering the ODC to make that SPO2 possible. That’s left for us to determine.
HbO.Dash simulated computation assumes the following fixed values for two of the less important parameters (background 2,3DPG [4.65 mM] and 37.5 C for temperature). Most often these assumptions are nominally misleading, but usually won’t play significant roles in masking oxygenation dysfunction unless massive transfusion is needed or complications arising from surgery and/or anesthesia unexpectedly occur. 2,3DPG is an organophosphate created in red blood cells during glycolysis, and its production increases with diminished peripheral O2 availability (e.g. hypoxemia, chronic pulmonary disease, anemia, and CHF). High levels shift the ODC rightward, while low levels associated with septic shock and hypophosphatemia can cause a leftward shift, again where the SPO2 for any given PaO2 value becomes artificially elevated. Likewise, CO2 can have a double edged effect on the ODC. We’ve already discussed its important Bohr effect from arterial blood pH changes that occur with PaCO2 variability. But CO2 accumulation also causes carbamino compounds to be generated through chemical interactions that once high enough can shift the ODC leftward, also falsely elevating the SPO2.
This is a lot of information to keep track of. Why should we be able to do this, or at the very least understand why it’s done? The reason ‘why’ becomes critically important once we acknowledge that a large number of bedside clinicians have allowed themselves to become dependent on monitor alarms (e.g. continuous pulse oximetry) to do their thinking for them. This is Threshold Science fallout! Our threshold alarms, as they are generally used today, are incapable of adequately detecting our RECC Types early. At times they are incapable of detecting our RECC Types at all. We discussed early in this chapter that most pulse oximetry monitors, even those used on GCF, are set to alarm once the SPO2 value dips below 90 %. This particular GCF practice continues to be associated with both alarm fatigue and poor opioid associated GCF outcomes, nor is it practiced universally across our nation. Dartmouth’s Hitchcock Medical Center has been deploying universal GCF pulse oximetry monitoring with alarm thresholds set at 80 %, and boasts no opioid associated deaths since 2007 without any nuisance alarms [42]. Nevertheless, 90 % thresholds account for the majority of GCF practices today because most individual institutions have not thought the practice consequences through properly, still mistakenly believing the SPO2 90 % threshold represents an unimpeachable standard of care. But if we believe ourselves to be clinicians expertly capable of managing patients receiving parenteral opioids, one of our skill sets should certainly be to reliably detect opioid associated CO2 narcosis (Type II PUHD) at its earliest (PaCO2 = 70 mmHg) when it can be easily corrected. In order to do this, we need to at minimum be aware of the fundamentals of respiration, including an appreciation for its complexity and potential Boobie Traps. We’ll now look again at our healthy 50 year old with normal lungs, but will make him a PACU patient with you in charge. We will also compare him to a healthy 30 year old and 75 year old in order to get you comfortable with the vagaries of respiratory complexity and opioid associated Type II PUHD influences. We will assume that this hypothetical PACU is at sea level for those of you clever enough to insist on this amount of granularity.
All three of your hypothetical patients go through operations where there is minimal blood loss but significant pain. For our first vignette, assume each is about to leave the PACU on room air, all are comfortable from appropriately administered intermediate opioids, all easily aroused with SPO2 of 92 % and breathing at 10/min. They have all been started on standard, demand only morphine PCAs, and will be monitored with continuous pulse oximetry once on the GCF. What might be your concerns? You certainly have done a nice job managing their pain, and there seems to be physiologic evidence that opioids are producing some respiratory depression along with high quality analgesia. Because these are otherwise healthy individuals, and you feel somewhat confident in the safety profiles of demand only PCAs, you shouldn’t feel a need to subject these patients to arterial sticks for obtaining blood gases. What you might want most is reassurance that should these patients become progressively more narcotized to the point of CO2 narcosis (PaCO2 = 70 mmHg), that this will be reliably detected on the GCF. You also know how busy the GCF can get and that your GCF oximeters alarm once SPO2 values breach 90 %, just like yours in the PACU. If you are sensitive to ACLS recommendations, stating severe arterial acidosis comprises pH<7.20 you might also want to keep these patients’ arterial pH above 7.25 (which can be done in the face of simple respiratory acidosis by limiting the maximum PaCO2 to 55 mmHg). So given all this, let’s have a look under the hood using mathematical models to project just how safe these patients will be on the GCF with continuous pulse oximetry surveillance.
Your 30 year old patient breathing room air will have a PAO2-PaO2 (A-a gradient) of 10 mmHg, your 50 year old a 15 mmHg gradient, and your 75 year old a 21 mmHg gradient. You can’t calculate precisely what your PAO2 will be because you don’t know what your PaCO2 is, but you do know you prefer the PaCO2 to not exceed 55 mmHg. So working backwards with the assumption that your 30 year old’s PaCO2 is 55 mmHg, his PAO2 calculates out to be 81 mmHg. Subtract his A-a gradient of 10 mmHg and his PaO2 would be 71 mmHg. This value correlates to a HbO.Dash SPO2 computation value of 91 %, 1 % beneath his current PACU SPO2 92 %, indicating at the moment a job well done since his PaCO2 must be somewhere beneath your 55 mmHg limit. But there’s not a lot of cushion! The HbO.Dash computation also tells you with appropriate recalibrations of his PAO2 that this patient’s PaO2 would need to drop to 67 mmHg to trigger a pulse oximeter’s alarm set for a 90 % SPO2 breach. It further quantifies that any such breach would correlate with a PaCO2 of 58 mmHg that calculates to a pH of 7.24, neither ideal but well beneath a PaCO2 of 70 mmHg where emergent, though relatively simple therapeutic interventions would be needed to bring ventilatory performance back in line.
Your 50 year old has a 15 mmHg A-a gradient, so working with the same assumptions above, his PaO2 drops to 66 mmHg. This correlates to a HbO.Dash SPO2 value of 89 %. Clearly, with a SPO2 value of 92 % on room air, his PaCO2 and pH will be within acceptable ranges. Additional computation shows us that the 90 % threshold pulse oximeter will begin alarming (SPO2 89 %) if his PaCO2 should climb to 55 mmHg. Your 75 year old has a 21 mmHg A-a gradient that drops his PaO2 to 60 mmHg at a PaCO2 of 55 mmHg. This correlates to a HbO.Dash SPO2 value of 87 %, leaving even more margin for safety regarding his pH with his PACU SPO2 being 92 %. In fact, the opioid respiratory depression I fictitiously assigned him doesn’t really align with his calculated clinically near normal PaCO2 of 46 mmHg, and pH of 7.34 that were worked out with multiple simulated HbO.Dash computation runs. But any PaCO2 higher than 50 mmHg would drop his SPO2 below 90 %, causing the GCF pulse oximeter to alarm needlessly, assuring unwanted nursing distraction should he choose to push his PCA demand button. Unfortunately, it also assures little rest for him and unsafe GCF conditions because of alarm fatigue. One way to circumvent this issue would be to provide supplemental oxygen, as we are accustomed to doing. But as will be demonstrated, this decision comes at a steep price.
Back again to your 30 year old patient. Instead of breathing room air in the PACU, he’s given 3 L/min O2 through nasal cannula. Let’s assume that this raises his FIO2 from .21 to .30, a commonplace assumption (.21 + (.03 × O2L flow/min)) when using this kind of oxygen delivery system [43]. Now his A-a gradient must be adjusted to accommodate this approximate .1 FIO2 addition, and the new gradient is estimated by adding an additional 5–7 mmHg to his original A-a gradient for each added .1 FIO2 increase. Because the FIO2 is now .3, his calculated PAO2 (assuming a PaCO2 of 55 mmHg) will now be 145 mmHg. So we subtract from this P AO2, his (age + 10)/4 + the additional 7 mmHg A-a adjustment, and his PaO2 approximates 128 mmHg. This PaO2 correlates to a HbO.Dash SPO2 above 98 %, meaning that at a pH of 7.26 and PaCO2 of 55 mmHg, the pulse oximeter will not indicate any sign of trouble. At a PaCO2 of 70 mmHg (pH of 7.16) his PAO2 calculates to 126.4 mmHg and his PaO2 with A-a adjustments approximates 109 mmHg. This yields a 95 % SPO2, a value that rarely earns more than cursory notice, yet now your patient is in serious trouble while probably appearing to be sleeping blissfully in no distress at all! Your 50 year old patient will have a near identical experience because with all the adjustments appropriate for his age, at a PaCO2 of 70 mmHg (pH of 7.16) his PaO2 calculates to 104 mmHg with a HbO.Dash SPO2 value again of 95 %! Your 75 year old doesn’t fair any better. At a PaCO2 of 70 mmHg (pH of 7.16) his PaO2 calculates down to 98 mmHg with his A-a adjustments, but the HbO.Dash SPO2 remains in the 95 % range, just a tad lower by fractions of a decimal point. Knowledge is always good, but can be frightening at times! Let’s see how things might improve as we attempt to limit your ‘low’ flows of supplemental O2, starting at 1 L/min.
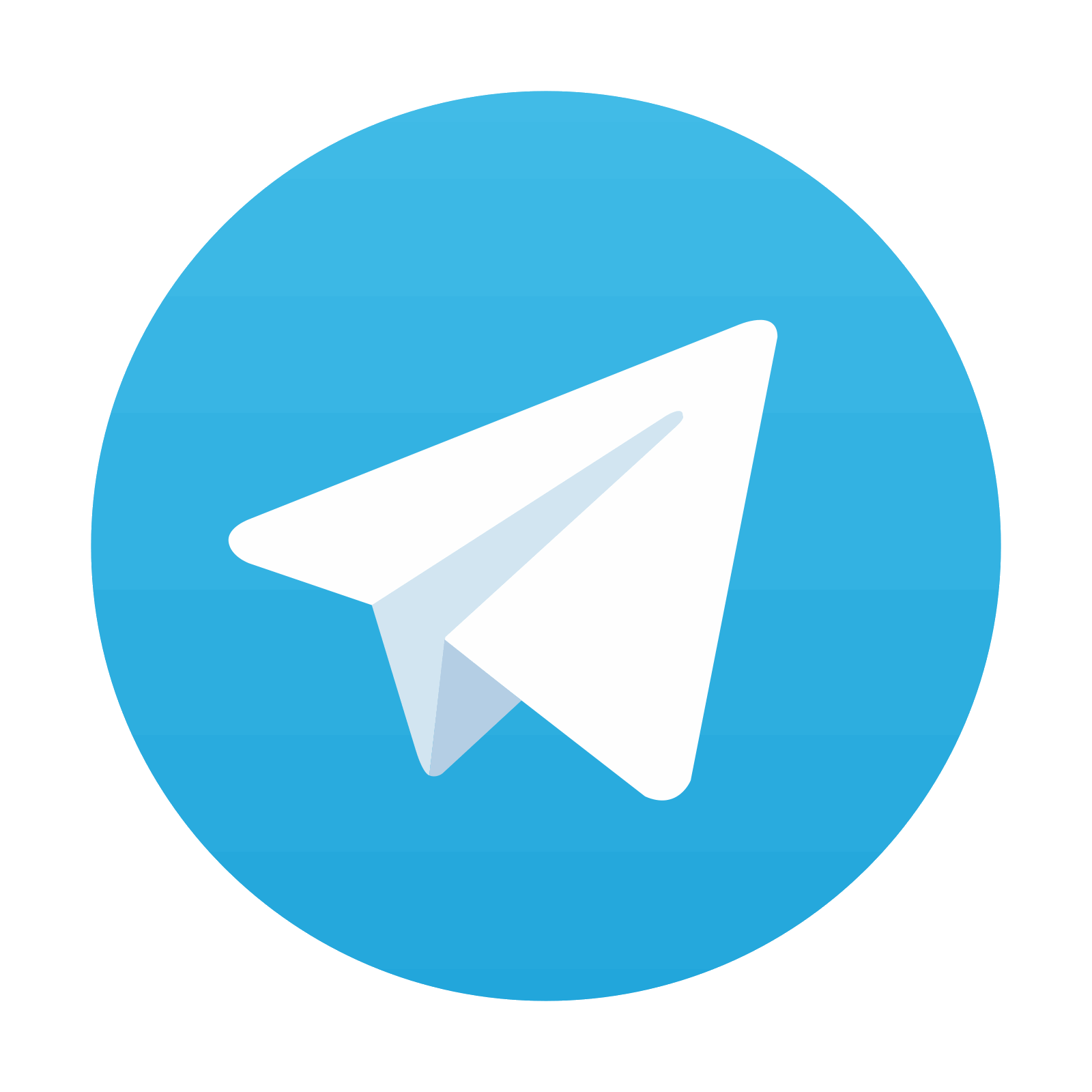
Stay updated, free articles. Join our Telegram channel
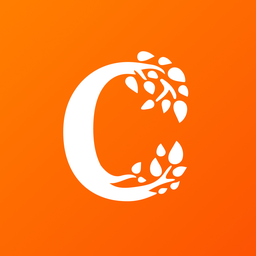
Full access? Get Clinical Tree
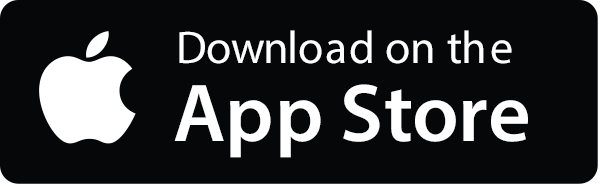
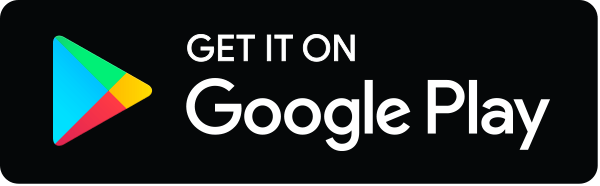