(7.1)
where p is the differential pressure across the length of the pore, D is the pore diameter, γ is the surface tension of the wetting liquid; and θ is the contact angle of the wetting liquid with the sample [130]. The volume of the liquid flowing out of the membrane is collected and weighed in an analytical balance. This volume corresponds to the flow-through pore volume within the scaffold. This technique works well for electrospun polymeric scaffolds; however, it may be replaced with simpler gravimetric analysis for other scaffold formations.
Polymer Hydrophilicty/Hydrophobicity
Polymer degradation by hydrolysis will be governed by the amount of water present in the formulation. The degradation rate is thus influenced by the hydrophobicity of the polymeric side chains and their ability to take on water. The hydrophobicity of the polymeric structure must strike a balance with the optimal hydrophobicity needed for protein adsorption and cellular attachment. In order for cells to adhere to a polymeric scaffold, the scaffold must be hydrophilic. However, many of the polymers utilized for scaffold fabrication are highly hydrophobic. Thus, surface modifications or pre-wetting the scaffold with a solvent is typically needed for successful cell adherence.
Testing for hydrophilicty/hydrophobicity is most commonly performed by calculating the contact angle of a water droplet on the surface of the material. The more hydrophobic the polymer is, the greater angle will be formed between the water droplet and the surface. For example, a material with a contact angle of 90° will be more hydrophobic compared to one with a contact angle of 30°. Contact angles may be measured using imaging and standard image analysis tools or physically measured with a goniometer.
Polymer Biomechanical Properties
The biomechanical properties of the polymer used in tissue engineering are an important factor in ensuring durability of the tissue engineered structure. The biomechanical properties must be understood at the time of implant and throughout degradation of the polymer as it is filled in with regenerated tissue. For example, if a polymer is utilized to repair a defect in a mineralized, load bearing tissue, such as bone, the tissue engineered polymer construct must be able to withstand the loads during healing. If a polymeric scaffold is used in a vascular graft repair, it must be able to withstand the shear stress environment under pulsatile flow. A clear understanding of the mechanical profile of the material and the mechanical environment at the location of the implant is necessary for a successful engineered tissue.
Common tests for biomechanical properties include traditional uniaxial testing, biaxial testing, three-point bending, compression, or torsion tests. In all of these modes of mechanical testing, the viscoelastic (or time dependent) nature of the polymer must be assessed. Biomechanical tests need to be performed on the polymer alone, the polymer + cells, and explanted tissues from animal studies. Each of these sample groups can be compared to the biomechanical properties of the native tissue that is to be replaced. These tests are often utilized in combination with finite element analysis and computational simulations to fully understand the biomechanical properties of the engineered tissue. Many experts in the field believe the mechanical tests to be the ultimate measure of a tissue engineered polymer. However, in vivo testing in relevant animal models is likely the best measure prior to use in patients.
Other Important Considerations in the Design of Biodegradable Polymers for Functional Tissue Replacement
Polymer scaffolds of conventional tissue engineering techniques must not only degrade at a kinetic rate appropriate for tissue replacement, the scaffolds must also serve as templates to engineer the proper cellular niche for cellular differentiation into a pro-regenerative phenotype. Growth factors or other additive factors may be added to the polymer scaffold for release. These growth factors or other drugs will provide a favorable cellular microenvironment and/or may serve to recruit cells to the site for regeneration. Growth factor or drug release is measured similarly to in vitro degradation rate tests as described above. Growth factor release may be assayed in sampling buffer from the scaffold at various time points via enzyme linked immunoabsorbant assay (ELISA). Many new advances in the synthesis of polymers as degradable cellular templates are being reported daily; however, few have made it to market.
As with any polymeric material that interfaces with the body, the acute and chronic toxicity of the polymer and its degradation byproducts must be taken into consideration. The best way to examine acute and chronic toxicity is through an animal model. For example, in the rat, acute toxicity would be measured within the first 24 h of implantation. Chronic toxicity would be assessed at 90 days post implantation, which corresponds to 10 % of the animal’s lifespan. Toxicity is commonly assessed by material tracking in vivo through X-ray, MRI, or other non-invasive in vivo imaging such as IVIS (Perkin Elmer). At the end of the time duration, histological analysis is performed to ensure that the material causes minimal cell death at the site of implantation. For a tissue engineered implant containing a polymeric biomaterial to be safe and effective, chronic toxicity must be eliminated.
Polymers for Engineering Complex Architectures Such as the Lung and Liver
Several different polymers are utilized in order to engineer tissues with complex tissue architectures and highly specialized cell function. The focus of this section will be on the lung and liver. Both the lung and liver are organs with high need of replacement due to fibrotic disease or other dysfunction. There is high unmet demand for donor lungs and livers. Tissue engineering strategies may either fully replace the lung or liver or may be employed in assist devices to replicate function until a donor organ is available. This section will highlight the course of research in polymeric based tissue engineering the lung and liver. The state of the art in tissue engineering these two organs has followed a similar course of development as follows: polymers for structured cellular transplantation, the use of combined synthetic and naturally-derived polymer approaches to large structures, the use of decellularized tissues, and the use of polymers in assist devices.
Polymer Sponges or Hydrogels for Structured Cellular Transplantation
The basic paradigm for engineering polymeric structures for cellular transplant in the lung and liver is to utilize a hydrogel or sponge structure with either adult differentiated cells or progenitor cells. In both the lung and the liver, these polymer-cell structures have shown great success in vitro. Additionally, in vivo animal studies show promising results of cellular survival and functional differentiation.
Although the liver has some regenerative ability, there is a large need for liver transplantation due to cirrhosis, drug toxicity, or congenital defect. Tissue engineering functional liver units has been a focus for many years as a potential source for liver replacement. The first examples of functional transplantable liver-like structures used prevascularized, non-degradable polyvinyl alcohol sponges to accommodate transplanted hep-atocytes with limited success [131, 132]. Modified gelatin sponges with lactobionic acid (MGLA) have also been used to support mouse hepatocyte growth [133]. Microhydrogels made of fibrinogen attached to poly(ethylene glycol) (PEG)-diacrylate side chains were used as a cell carrier for intravascular transplantation of hepatocytes in a rat model [134]. Another naturally derived material, silk fibroin, has been utilized in several tissue engineering approaches. Silk fibroin is a naturally-derived material which has high molecular weight organic polymers characterized by repetitive hydrophobic and hydrophilic peptide sequences. Silk fibroin assembles into regular structures during materials formation and can be considered as nature’s equivalent to synthetic block copolymers [135]. Due to its polymeric nature, silk fibroin has been utilized in a cryogel to support hepatocyte growth with aims for future transplantation [136]. A cryogel is a porous hydrogel formed from polymerization under low temperature conditions. The ice crystals that form allow for highly interconnected pores within the hydrogel. Preparation and assessment of a gelatin hydrogel with methacrylated crosslinking is shown in Fig. 7.1.
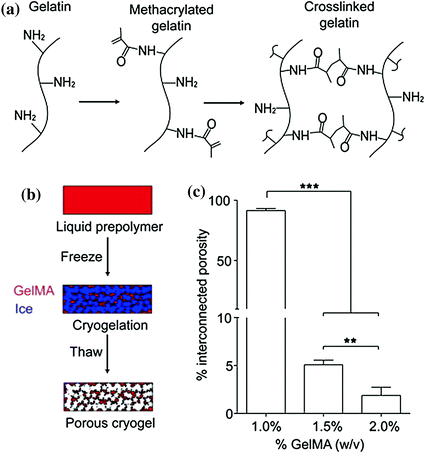
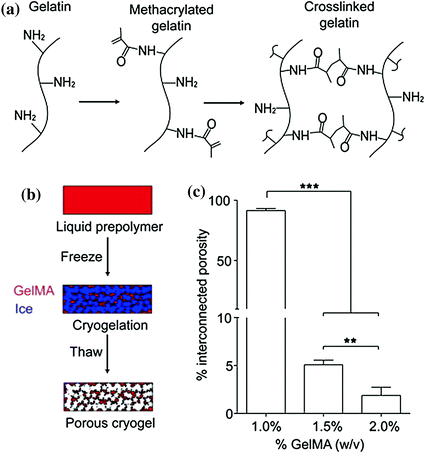
Fig. 7.1
Preparation and assessment of gelatin cryogel. a Schematic of GelMA synthesis and crosslinking. Pendant methacrylate groups are added primarily to the free amines of gelatin by reaction with methacrylic anhydride. Free radical polymerization results in crosslink formation between methacrylate groups. b Cryopolymerization of methacrylated gelatin. Freezing of methacrylated gelatin in the presence of radical initiators (APS and TEMED) allows polymerization to occur in the partially frozen state (cryopolymerization). Ice crystals formed during the freezing process and thawing after cryopolymerization results in the formation of a hydrogel with micron-scale pores. c Volume of interconnected pores in gelatin cryogels (normalized to total gel volume). Values represent mean and standard deviation (n = 10). Data were compared using ANOVA with Bonferroni’s post-hoc test (**p < 0.01, ***p < 0.001). Reproduced from [163]
In the lung, similar approaches have been employed focusing on naturally-derived polymers alone or in combination with synthetic scaffolds. Gelfoam sponges are commercially available surgical devices that are derived from porcine skin gelatin (Pfizer.com). Gelfoam sponges are water-insoluble and capable of absorbing up to 45 times their weight (Pfizer). Gelfoam sponges have been utilized to grow lung organotypic structures in vitro [137, 138]. Additionally, Gelfoam sponges seeded with fetal rat lung cells were viable in the adult animal lung for up to 35 days with neovascularization apparent [139]. Other hydrogels with natural and synthetic components have been used to engineer pulmonary cell structures. The natural components of these are typically either gelatin or Matrigel. Gelatin is a low-cost, non-immunogenic natural material derived from collagen. Matrigel is a product from Corning Life Sciences that is a gelatinous extracellular matrix mixture made from mouse sarcoma cell secreted matrix. These two naturally-derived materials have been used in vitro and in vivo animal models extensively. Additionally, gelatin has been used in numerous human applications, which makes it an appealing polymer for new tissue engineered products. Gelatin in the form of a three-dimensional microbubble scaffold was used to provide the proper cellular microenvironment for differentiation of mouse pulmonary stem cells into alveolar pneumocytes [140]. Similarly, hydroxyethyl methacrylate-alginate-gelatin (HAG) hydrogels have been employed as three-dimensional structures for lung epithelial cell growth [141]. Additionally, three-dimensional structures for alveolar cell growth using matrigel hydrogel and synthetic polymer scaffolds of poly-lactic-co-glycolic acid (PLGA) and poly-l-lactic-acid (PLLA) fabricated into porous foams and nanofibrous matrices have been used successfully in vitro [142]. Engineering the cellular microenvironment for progenitor cell differentiation will likely continue to be the focus of new polymer formulations in lung and liver cell delivery.
Naturally-Derived and Synthetic Polymer Strategies for Larger Pulmonary and Hepatic Structures
While hydrogels or sponges have been examined for cell transplant for the parenchyma of the liver or lung, more traditional tissue engineering approaches have been utilized for some of the larger structures such as bile ducts, trachea, or bronchioles. These traditional tissue engineering approaches utilize scaffold materials made from naturally-derived and/or synthetic polymers and have been implanted with some success in vivo in both humans and in animal models.
In the liver, there have been few attempts to re-create functional liver structures using polymeric biomaterials in the context of engineering the bile duct or liver capsule. Three dimensional stacked polycarbonate membranes with hepatocytes showed functional liver units in vitro [143]. Functional liver units were also observed using micropatterned Poly(ethylene glycol)-poly(dl-lactide) and lactosylated poly(dl-lactide) electrospun fibrous mats [144]. For larger structures, like the bile duct or vascular structures, polymers may be formed into sheets and then tube structures. The bile duct was successfully replaced in an animal study using polycaprolactone and polylactic acid reinforced with polyglycolic acid fibers in a tube structure [145]. Continuing advances in synthetic polymer processing, such as three-dimensional printing and micropatterning, will improve the field of engineering larger liver structures such as the bile duct.
In the lung, tissue engineering strategies have been employed to engineer larger airway structures such as the trachea, main stem bronchi, and bronchiole. In 2008, the first transplantation of a tissue-engineered trachea in a human being was done to replace an end-staged left main bronchus with malacia in a 30-year-old woman. The implanted trachea was engineered from a decellularized cadaveric trachea seeded with autologous epithelial cells and mesenchymal stem cell-derived chondrocytes [146]. Five years post implantation, the tissue engineered trachea remained viable and patent with stenting needed in the native trachea near the implant [147]. This initial success advanced the field of clinical applications of tissue engineered airways (Fig. 7.2).
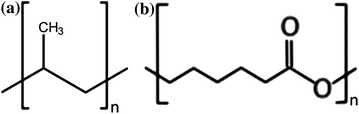
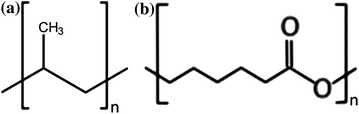
Fig. 7.2
Chemical formulas for a Polypropylene and b Polycaprolactone
New advances and improvements on tissue engineered trachea involve synthetic and natural combination polymer strategies. Polypropylene meshes with collagen and/or poly(l-lactic-acid-co-e-caprolactone) coating have been used in an animal model for replacement of the left main stem bronchi [148, 149]. Greater success was achieved utilizing the poly (l-lactic-acid-co-e-caprolactone) coating for epithelial regeneration. Other success has been reported in a rabbit model where tissue engineered tracheas were formed from articular cartilage matrix and chondrocytes [150]. New advances in 3D printing polymers have been harnessed for applications to the airway. A half-pipe polycaprolactone 3D printed trachea seeded with mesenchymal stromal cells in a fibrin matrix was implanted into a rabbit model with initial success of regenerated epithelium [151]. Tissue engineering polymer approaches for the large airways have gained momentum due to the ease of fabrication and implantation. However, smaller airways, termed bronchioles, have been attempted for in vitro understanding of small airway diseases such as asthma with the eventual goal of a functional tissue engineered lung. For the bronchiole, a type I collagen gel seeded with fibroblasts, epithelial cells, and airway smooth muscle cells was engineered utilizing a pulsatile flow bioreactor [152]. Similarly, another study cites tubular bronchiole structures engineered using airway smooth muscle tubular structure collagen pulsatile flow [153]. The engineering of these smaller airway structures highlights the need for advances in bioreactor technology to go hand in hand with the advances in polymeric biomaterials. A true tissue engineered lung will likely utilize a combination approach of polymeric materials, the proper cell choices, and proper in vitro conditioning.
Vascularization and the Mechanical Environment
Vascularization is often the limiting factor in tissue engineering complex three dimensional tissues for lung and liver replacement. Both hepatocytes and pulmonary epithelium have high oxygen needs, so the design of polymeric structures must allow for a highly vascularized system. Furthermore, the blood supply is crucial in both of these systems to maintain functionality. The liver’s main function is to filter the blood coming from the digestive tract, and the lung’s main function is to allow for gas exchange in the blood to occur. This functionality, while seemingly obvious to include, is non-trivial when engineering three dimensional structures for replacement of liver and lung. Several strategies have been employed to improve oxygenation of cells grown in vitro including the addition of perfluorcarbons and the bubbling of oxygen through the culture media.
In addition to the flows from the vasculature, the mechanical environment of the three-dimensional construct must be taken into account. When engineering a portal triad for the liver, the shear forces in the bile duct must also be considered in the design. Furthermore in the lung, a very complex mechanical environment exists as the lung is constantly distended and relaxed. The polymeric structures used to engineer the lung must have large elastic recoil in order to withstand this repeated deformation. Due to the complex mechanical environment and vascularization structure of the lung and liver, decellularized organs may provide the best natural polymeric scaffolding material.
Decellularized Organs
Decellularized organs from human cadavers or animal sources provide the structural architecture necessary for complex three dimensional tissues. Key natural polymers, proteins, and structural components remaining in decellularized organs are collagen types I–IV, elastin, fibronectin, and laminin. Depending on the mode of decellularization (as described in Sect. “Biologically Generated Polymers”), many active growth factors and cytokines may also be present in the matrix.
Lung decellularization has had successes in animal implantation [154]. Several other studies have examined effects of decellularization processes on matrix architecture and strength [155, 156]. Lung decellularization leaves the complex architecture of the lung airways and alveoli with the vascular network available for repopulation with endothelial cells (Fig. 7.3). Repopulation of decellularized lung matrix with progenitor cells and subsequent differentiation into lung phenotypes shows that the decellularized lung matrix provides a hospitable environment for regeneration [157]. Although there has been much progress in the field of decellularized lung scaffolds, it remains unclear what the proper cellular choices are for recapitulating proper lung function and air-tight gas exchange.
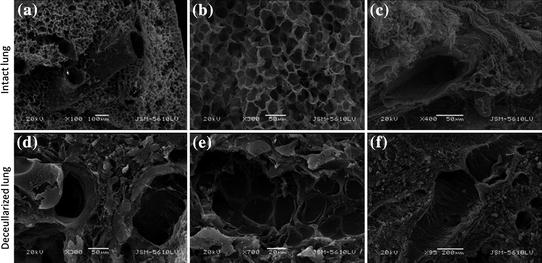
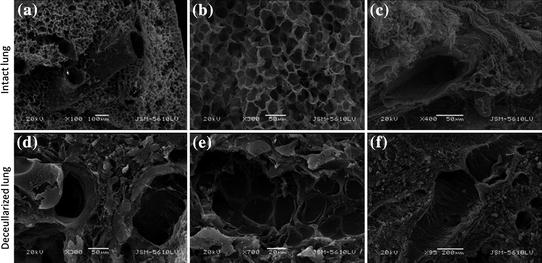
Fig. 7.3
Intact and decellularized mouse lung shows retention of pulmonary structures. a and d show larger airways, b and e show alveoli, c and f show blood vessels
Liver structure/function relationship is paramount in engineering hepatic units. To retain the complex structure/function relationship, decellularized livers have also been utilized for repopulation and transplantation into in vivo models [158, 159]. Using the decellularized livers as scaffolds causes the proper cellular spatial distribution that is so difficult to achieve using other polymeric scaffold fabrication techniques. As with the lung, similar issues remain to be worked out in whole organ liver tissue engineering. These issues include clotting, cell choice, cell fate, and long term functionality of the organ.
Assist Technologies
Due to the complexity and remaining issues in engineering replacement organs for lungs and livers, “lab on a chip” approaches have been utilized to engineer assist devices. The assist devices can provide essential replacement of function that is missing even in decellularized organs. These assist devices are meant to perform the function of the lung or liver as either a bridge to transplant or as a permanent assist technology. The majority of assist devices are fabricated using soft photolithography or three dimensional printing. In the former, polydimethysiloxane (PDMS) is typically the polymer of choice because it can be poured into a photolithographic mold. The PDMS is often coated with a naturally-derived extracellular matrix protein such as collagen or fibronecin. Cells are then placed within the channels to perform function [160, 161]. With 3D printing, the polymer choice is broadened such that any polymer that can be put into solution at a viscosity matching the printer nozzle may be utilized. For the liver, polymers have been used as the structural components of assist devices as well as to perform function by capturing toxins (Fig. 7.4). In the lung, endothelial cells and ECM proteins have been added to polymeric tubes for enhanced gas exchange in extracorporeal membrane oxygenation devices [162]. There will continue to be a future push toward incorporating tissue engineered external devices to avoid coagulation and better prognosis for assist or hybrid technologies.


Fig. 7.4
Bio-inspired 3D liver detoxification device. Polydiacetylene nanoparticles (green) are installed in poly(ethylene glycol) diacrylate hydrogel matrix (grey) with liver-mimetic 3D structure fabricated by 3D printing. The nanoparticles attract, capture and sense toxins (red), while the 3D matrix with modified liver lobule structure allows toxins to be trapped efficiently. This biomimetic 3D detoxifier has promising clinical application for detoxification by collecting and removing toxins. Reproduced from [164]
References
1.
Leahy, J.C., Hukins, D.W.: Viscoelastic properties of the nucleus pulposus of the intervertebral disk in compression. J. Mater. Sci. Mater. Med. 12, 689–692 (2001)
2.
Anderson, J.M., Andukuri, A., Lim, D.J., Jun, H.-W.: Modulating the gelation properties of self-assembling peptide amphiphiles. ACS Nano 3, 3447–3454 (2009). doi:10.1021/nn900884n
3.
Holmes, T.C., de Lacalle, S., Su, X., et al.: Extensive neurite outgrowth and active synapse formation on self-assembling peptide scaffolds. Proc. Natl. Acad. Sci. USA 97, 6728–6733 (2000)
4.
Mujeeb, A., Miller, A.F., Saiani, A., Gough, J.E.: Self-assembled octapeptide scaffolds for in vitro chondrocyte culture. Acta Biomater. 9, 4609–4617 (2013). doi:10.1016/j.actbio.2012.08.044
5.
Banwell, E.F., Abelardo, E.S., Adams, D.J., et al.: Rational design and application of responsive alpha-helical peptide hydrogels. Nat. Mater. 8, 596–600 (2009). doi:10.1038/nmat2479
6.
Jun, H.-W., Paramonov, S.E., Dong, H., et al.: Tuning the mechanical and bioresponsive properties of peptide-amphiphile nanofiber networks. J. Biomater. Sci. Polym. Ed. 19, 665–676 (2008). doi:10.1163/156856208784089625
7.
Kopecek, J., Yang, J.: Peptide-directed self-assembly of hydrogels. Acta Biomater. 5, 805–816 (2009). doi:10.1016/j.actbio.2008.10.001
8.
Chau, Y., Luo, Y., Cheung, A.C.Y., et al.: Incorporation of a matrix metalloproteinase-sensitive substrate into self-assembling peptides—a model for biofunctional scaffolds. Biomaterials 29, 1713–1719 (2008). doi:10.1016/j.biomaterials.2007.11.046
9.
Han, S., Cao, S., Wang, Y., et al.: Self-assembly of short peptide amphiphiles: the cooperative effect of hydrophobic interaction and hydrogen bonding. Chem. Weinh. Bergstr. Ger. 17, 13095–13102 (2011). doi:10.1002/chem.201101970
10.
Xu, X.-D., Jin, Y., Liu, Y., et al.: Self-assembly behavior of peptide amphiphiles (PAs) with different length of hydrophobic alkyl tails. Colloids Surf. B Biointerfaces 81, 329–335 (2010). doi:10.1016/j.colsurfb.2010.07.027
11.
Stendahl, J.C., Rao, M.S., Guler, M.O., Stupp, S.I.: Intermolecular forces in the self-assembly of peptide amphiphile nanofibers. Adv. Funct. Mater. 16, 499–508 (2006). doi:10.1002/adfm.200500161
12.
Löwik, D.W.P.M., Shklyarevskiy, I.O., Ruizendaal, L., et al.: A highly ordered material from magnetically aligned peptide amphiphile nanofiber assemblies. Adv. Mater. 19, 1191–1195 (2007). doi:10.1002/adma.200602295
13.
Feng, Y., Taraban, M., Yu, Y.B.: The effect of ionic strength on the mechanical, structural and transport properties of peptide hydrogels. Soft Matter. 8, 11723–11731 (2012). doi:10.1039/C2SM26572A
14.
Ramachandran, S., Taraban, M.B., Trewhella, J., et al.: Effect of temperature during assembly on the structure and mechanical properties of peptide-based materials. Biomacromolecules 11, 1502–1506 (2010). doi:10.1021/bm100138m
15.
Yunoki, S., Ohyabu, Y., Hatayama, H.: Temperature-responsive gelation of type I collagen solutions involving fibril formation and genipin crosslinking as a potential injectable hydrogel. Int. J. Biomater. (2013). doi:10.1155/2013/620765
16.
Alamein, M.A., Stephens, S., Liu, Q., et al.: Mass production of nanofibrous extracellular matrix with controlled 3D morphology for large-scale soft tissue regeneration. Tissue Eng. Part C Methods 19, 458–472 (2012). doi:10.1089/ten.tec.2012.0417
17.
Pham, Q.P., Sharma, U., Mikos, A.G.: Electrospinning of polymeric nanofibers for tissue engineering applications: a review. Tissue Eng. 12, 1197–1211 (2006). doi:10.1089/ten.2006.12.1197
18.
Baker, B.M., Gee, A.O., Metter, R.B., et al.: The potential to improve cell infiltration in composite fiber-aligned electrospun scaffolds by the selective removal of sacrificial fibers. Biomaterials 29, 2348–2358 (2008). doi:10.1016/j.biomaterials.2008.01.032
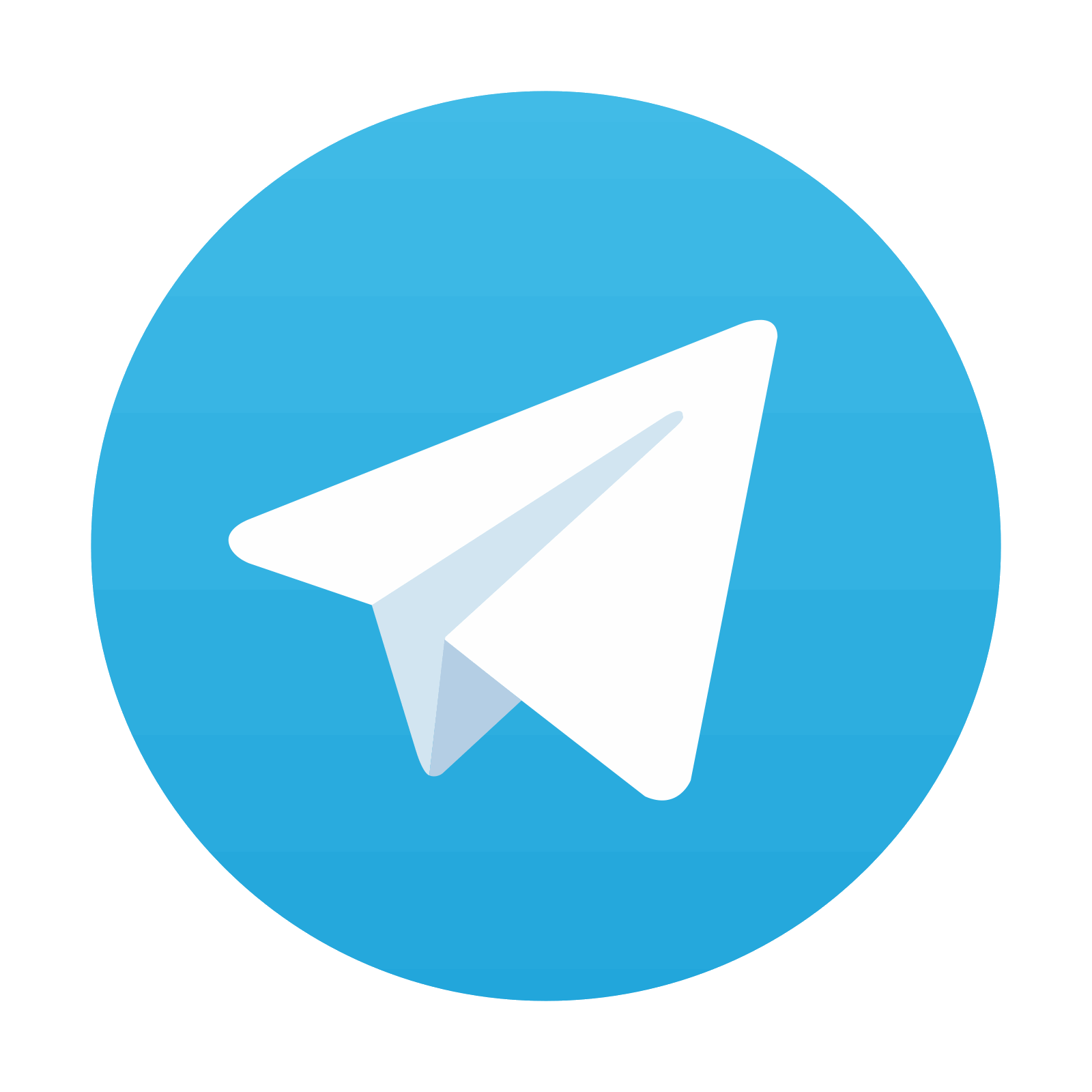
Stay updated, free articles. Join our Telegram channel
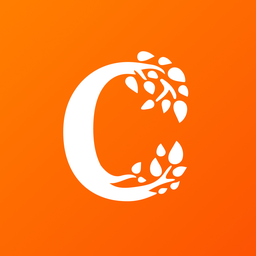
Full access? Get Clinical Tree
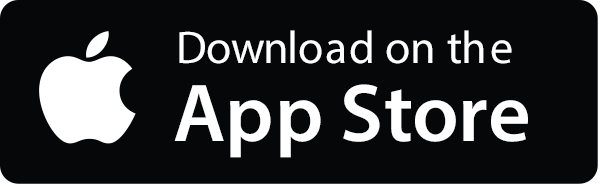
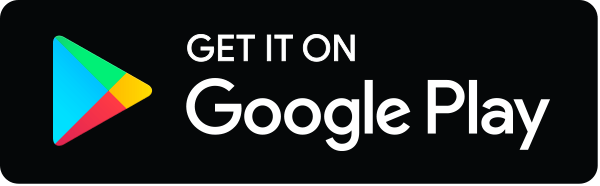