Fig. 10.1
Representation of EPR effect
This occurs because of the abnormalities of tumor vasculature, namely hypervascularization, aberrant vascular architecture, extensive production of vascular permeability factors and lack of lymphatic drainage [4], which stimulate the extravasation of nanoparticles and the accumulation of drugs in the tumor interstitium, improving their therapeutic efficacy and reducing harmful non-specific side effects [5]. In addition, nanosized materials administered intravenously (i.v.) escape renal clearance due to their large size.
When loaded with imaging agents, these kinds of systems offers opportunities to exploit optical imaging or MRI in cancer imaging, and guided hyperthermia therapy.
In literature, several examples of nanoparticulate systems with a variety of composition for cancer treatment are reported, including liposomes, polymeric nanoparticles, gold nanoparticles, paramagnetic nanoparticles, and so on. Among others, polymeric materials offer unique advantages such as the easy preparation, mechanical strength, versatility and the possibility to easily modulate their physico-chemical properties.
This chapter is focused on the recent advances in the design and synthesis of polymeric nanosized materials in cancer therapy, diagnosis and theranostics.
Nano-sized Polymers in Therapeutic
The combination of controlled release technology and targeted drug delivery may provide a more efficient and less harmful solution to overcome the limitations found in conventional chemotherapy. Recent interest has been focused on developing nanoscale delivery vehicles based on synthetic or natural polymers capable of controlling the release of chemotherapeutic agents directly inside cancer cells [6–8]. Depending from the chemical-physical properties, these systems can release encapsulated drugs through surface or bulk erosion, diffusion, or swelling followed by diffusion, in a time- or condition-dependent manner. Moreover, the release may be constant over a long period, or it may be triggered by the environment or other external events [9]. In general, controlled-release polymer systems can provide drug levels in the optimum range over a longer period of time than other drug delivery methods, thus increasing the efficacy of the drug and maximizing patient compliance.
Generally, the exploited processes to deliver drug-encapsulated in nanoparticles to cancerous tissues are passive and active targeting. As described above, the first approach uses the unique properties of the tumor microenvironment, such as the leaky tumor vasculature, which is highly permeable to macromolecules and the dysfunctional lymphatic drainage system, which results in enhanced fluid retention in the tumor interstitial space [10]. As before explained, the tumor-specific deposition, also known as the EPR effect, occurs as the nanoparticles extravasate out of tumor microvasculature, leading to an accumulation of drugs in the tumor interstitium. The extent of nanoparticles extravasation depends on the size of open interendothelial gap junctions and trans-endothelial channels. In general, particles with diameters less than 200 nm are the most effective for extravasating tumor microvasculature [11, 12]. Besides the size particle modulation, another strategy to obtain a passive drug targeting consists in designing engineered materials able to program the drug delivery in response to the variations of some parameters of the surrounding environment. The responsiveness or smartness of these systems refers to their ability to receive, transmit a stimulus, and respond with a useful effect. Typical stimuli are the variations of pH, temperature, redox potential, light, magnetic field, and concentrations of electrolytes or glucose. For example, the acidic environment of cancerous tissues (pH 6.5–7.2) [13, 14], endosomes (pH 5.0–6.5) [15] and lysosomes (pH 4.5–5.0), as well as the higher concentration of redox species in the intracellular space of tumor cells [16] and the enhanced temperature in cancer tissue, can be exploited in anticancer drug delivery and intracellular drug delivery.
The responses of the so-called “smart” drug delivery vehicles can be dissolution/precipitation, swelling/collapsing, hydrophilic/hydrophobic transition, bond cleavage, degradation, drug release, and so on. The second strategy to specifically deliver the drug in tumor site is active targeting. Active tumor targeting is typically achieved by conjugating a targeting molecule, that can recognize and bind to specific ligands that are unique to cancer cells, on the particle surface. In the following sections, the main classes of polymeric nanosized materials to be used in targeted cancer therapy are described.
Dendrimers
Dentritic polymers, or dendrimers, are synthetic, highly branched, spherical, monodispersed macromolecules with an average diameter of 1.5–14.5 nm [17, 18]. The typical dendrimer structure consists of an initiator core, highly branched layers composed of repeating units, and multiple active terminal groups. They can be synthesized with either divergent methods (outward from the core) or convergent methods (inward towards the core). Tomalia was the first to synthesize the 3D (polyamidoamine) dendrimers using divergent methods [19]. These dendrimers contain tertiary amines that allow the binding of a number of molecules. In the convergent approach, established by Frechet [20], the dendrimer results from the generation of monomers added to a main core. Dendrimers have emerged as an important class of drug complexing/encapsulating systems and in literature several examples of the employment of these materials as drug delivery vehicles have been reported, although one limitation lies in the effort of controlling the rate of drug release. The encapsulated, complexed, drugs tend to be released rapidly (before reaching the target site) and in the dendrimer-drug conjugates, it is the chemical linkage that controls the drug release. However, dendrimers offer several advantages as drug carriers targeting cancer. One major advantage is their surface functionality providing the selective coupling of imaging agents, targeting ligands and/or other components to increase tumor specificity [1]. In a work of 2002, Henrik et al. [21] proposed biodegradable polyester dendrimers based on 2,2-bis(hydroxymethyl)-propanoic acid monomers for intracellular release of DOX after hydrolysis of the hydrazone linkage. Moreover, DOX was conjugated to a biodegradable dendrimer with optimized blood circulation time through the careful design of size and molecular architecture Specifically, the DOX-dendrimer controlled drug-loading through multiple attachment sites, solubility through PEGylation, and drug release through the use of pH-sensitive hydrazone dendrimer linkages. In culture, DOX dendrimers were N10 times less toxic than free DOX toward colon carcinoma cells. Upon intravenous administration to tumor bearing mice, tumor uptake of DOX-dendrimers was nine-fold higher than intravenous free DOX and caused complete tumor regression and 100 % survival of the mice after 60 days [22].
Nanogels
Nanogels are nanosized hydrogel particles formed by physical or chemical cross-linked polymer networks. The main characteristic of these systems consists in their ability to retain considerable amount of water, but also the biocompatibility, the stability in aqueous media and the versatility in release drugs in a controlled manner are common properties of this class of materials. Nanogels made from synthetic polymers offer well-defined morphologies that can be customized to gel networks with biocompatible and degradable properties. Bisht et al. [25] synthesized polymeric nanoparticle encapsulated formulation of curcumin—nanocurcumin—utilizing the micellar aggregates of cross-linked and random copolymers of NIPAAM, with VP and PEG-A. Further, the mechanism of action of nanocurcumin on pancreatic cancer cells mirrors that of free curcumin, including induction of apoptosis, blockade of nuclear factor kappa B activation, and downregulation of pro-inflammatory cytokines (i.e. IL-6, IL-8 and TNF-α). Nanocurcumin provides an opportunity to expand the clinical repertoire of this efficacious agent by enabling soluble dispersion.
Nanogel networks formed of stimuli responsive units extensively regulate the drug release profile and have found large application in delivery of anticancer drugs. Nanogels generated with polymer chains containing ionizable repeating groups are suitable for pH-dependant release [26]. The construction of nanogel networks with P(AA/MAA) units and PNIPAAM chains underlies a rapid increase in the hydrophilicity LCST of the copolymer at all the pH ranges, particularly pH < 5 [27]. Polyampholytic or zwitterionic polymeric gel particles have also received priority owing to their interior structural features. These features enable a response under all pH conditions owing to their effectiveness at a wide range of isoelectric points [28]. MMA diethyl acrylate-diethy phthalate nanogels stabilized by PEGMEM resulted in varied sizes under different pH conditions, with size following an order of pH 9 > 2 > 5 [29].
Polymeric Micelles
Polymeric micelles result from the auto assembly of di-block amphiphilic copolymers and are composed of two separated functional segments: inner core and outer shell [30]. The outer shell controls the in vivo pharmacokinetic behavior, while the inner core is responsible for drug loading capacity, stability and drug release behavior. The suitable PM size, too large for extravasation from normal vessel walls and renal excretion, and too small for extravasation from tumor blood vessels, combined with the pathophysiological characteristics of solid tumor tissues, hypervascularity, incomplete vascular architecture, secretion of vascular permeability factors and absence of effective lymphatic drainage leads to EPR effect in solid tumors [4, 31] and warrants the passive targeting of these systems.
Biocompatible, targeted sterically stabilized micelles have been used as nanocarriers for camptothecin, a topoisomerase I inhibitor used in cancer therapy. Moreover, stealth micelle formulations have stabilizing PEG coronas to minimize opsonization of the micelles and maximize serum half-life. Currently, SP1049C, NK911, and Genexol-PM have been approved for clinical use [32]. SP1049C is formulated as DOX-encapsulated pluronic micelles. NK911 is DOX-encapsulated micelles from a copolymer of PEG-DOX-conjugated poly(aspartic acid), and Genexol-PM is a paclitaxel-encapsulated PEG-PLA micelle formulation. Polymer micelles have several advantages over other drug delivery systems, including increased drug solubility, prolonged circulation half-life, selective accumulation at tumor sites, and lower toxicity.
Polymersomes
Ps are vesicular systems made from synthetic amphiphilic block copolymers [33, 34] that are organized to form hollow spheres containing an aqueous solution in the core surrounded by a bi-layer membrane. The bi-layer membrane is composed of hydrated hydrophilic coronas both at the inside and outside of the hydrophobic middle part of the membrane separating and protecting the fluidic core from the outside medium. Compared to polymeric micelles, the main advantages of these systems is the possibility to load hydrophilic, hydrophobic or amphiphilic compounds, exploiting the aqueous core or the membrane bilayers. The aqueous core can be utilized for the encapsulation of therapeutic molecules such as drugs, enzymes, other proteins and peptides, and DNA and RNA fragments [35–37]. On the other hand, the membrane can be considered as a reservoir system for both hydrophobic [38, 39], and amphiphilic (i.e. octadecyl rhodamine B [40, 41]) molecules similar to cell membranes, which incorporate cholesterol and membrane proteins.
These properties make polymersomes very attractive materials for various applications in drug delivery, biomedical imaging and diagnostics [42].
In principle, drug release from Ps is governed by the diffusion of the drug through the membrane [43], but many examples of smart Ps, in which the physical-chemical properties of the starting block copolymers are modulated to respond to external stimuli (i.e. pH, temperature, redox conditions, light, magnetic field, ionic strength and concentration of glucose), are reported in literature and proposed in cancer therapy.
Ps based on PEGPTMBPEC were reported by Chen et al. and in vitro studies demonstrated that the release of paclitaxel as well as DOX from these Ps was faster at mildly acidic conditions than at physiological pH due to the faster degradation of PTMBPEC at mildly acidic conditions [39].
The occurrence of oxidation-reduction (redox) reactions in the body has also been reported as a means to control spatial drug release in the body [44, 45]. Oxidative conditions exist in extracellular fluids and inflamed or tumor tissues, while intracellular compartments are known to be reductive [46, 47]. Hubbell and co-workers developed oxidation responsive Ps based on PEG-PPS-PEG [48]. The hydrophobic PPS was oxidized and transformed within 2 h into hydrophilic poly(sulfoxides) and poly(sulfones) upon exposure to hydrogen peroxide in the (GOx)/glucose/oxygen system, leading to destabilization of the vesicular structure. Reduction-sensitive disulfide block copolymer, PEG-SS-PPS was used to prepare Ps that can protect therapeutics in the extracellular environment but releasing their contents within the early endosome when the Ps are taken up by cells [49].
Polymeric Nanoparticles in Diagnostic
The conventional imaging agents currently used in clinics for diagnosis suffer from disadvantages including the non-specificity, in vivo instability and toxicity. For example, imaging molecules such as the fluorophores compounds, including blue, green, or red fluorescence dyes with visible range (400–600 nm), have significant limitations such as tissue auto-fluorescence and light absorbing components [hemoglobin, deoxyhemoglobin (max. abs. 560 nm), and water] [50].
Consequently, delivery of low concentrations of contrast agents to region of interest affects image quality. In addition, they have limited chemical modification sites without significantly changing their biological activities. Due to their enormous versatility, nanoparticulate systems offer multifunctional capabilities to encapsulate and transport high concentrations of imaging probes selectively to diseased site inside the body.
Polymer-based imaging probes have a large surface areas (easy modification with a wide range of imaging moieties), improved plasma half-lives and stability, less toxicity, and improved targeting.
They help the detection process of tumor cells by concentrating and protecting the marker from degradation, in order obtain a more sensitive analysis.
Currently, the modalities available for imaging of nanoparticles include optical imaging, MRI, nuclear imaging, computed tomography, and ultrasound [51].
Optical imaging, which includes fluorescence and bioluminescence detection, and radionucleotide-based imaging are both very sensitive but typically have a resolution greater than several millimeters. This can be limiting, especially in small animal models in which higher resolution is more informative. Optical imaging is also restricted to a depth of only several centimeters, and quantification of the signal can be problematical due to significant tissue absorption of the signal. By contrast, MRI and computer tomography are much less sensitive but demonstrate a resolution less than 1 mm. MRI can image deep tissues and has the added advantage of not exposing patients to radiation. However, data acquisition is slow compared with other approaches [52].
For each of these imaging modalities, novel nanoparticles have been developed that can enhance tissue contrast or can identify specific biological changes.
Streptavidin-coated fluorescent polystyrene nanospheres [Fluospheres® (green fluores cence) and TransFluospheres® (red fluorescence)] were used in single color flow cytometry to detect the EGFR on A431 cells (human epidermoid carcinoma cells) [53]. The results showed that the fluorescent nanospheres provided a sensitivity 25-fold that of the conjugate streptavidin–fluorescein. The encapsulation of fluorescent markers resulted in objects that were brighter and more concentrated than when simple conjugates of single dyes were used. The same fluorescent nanoparticles were used in combination with R-Phycoerythrin (R-PE, reagent for flow cytometry) in multicolor flow cytometry, enabling the concomitant detection of the CD3 and CD4 receptors on JURKAT cells (human acute T-cell leukemia cells) [53].
Another approach consists in functionalizing nanoparticles with target-specific biomolecules for controlling the navigation under in vivo conditions.
Weissleder and colleagues [54] developed a method to image tumor-associated lysosomal protease activity in a xenograft mouse model in vivo using autoquenched NIRF probes. NIRF probes were bound to a long circulating graft copolymer consisting of poly-L-lysine and methoxypolyethylene glycol succinate. Following intravenous injection, the NIRF probe carrier accumulated in solid tumors due to its long circulation time and leakage through tumor neovasculature.
Moreover, multimodal polymeric micelles have been developed for the visualization of cancer cells, known to overexpress a specific receptor, by both optical and nuclear techniques [55]. To achieve active targeting, specific peptide sequences were conjugated to nanoparticles, increasing the specificity of tumor imaging [56].
Moreover, Yang et al. [57] developed PEG-coated micelles with embedded near-infrared fluorescent dye for dual optical and nuclear imaging applications, showing a prolonged blood residence and effective accumulation inside solid tumors in mice. In another work, polymeric NPs containing the fluorogenic probe Cy5.5 and the dark quencher BHQ-312, linked together by a peptide sequence specific for a MMP for in vivo tumor imaging were developed by Lee and co-workers [58]. MMPs are a family of zinc-dependent proteins involved in inflammatory diseases and cancer progression. When these NPs are exposed to the specific MMP, fluorescence emission of Cy5.5 occurs, due to the enzymatic cleavage of the peptide bond between Cy5.5 and the quencher. The specificity of tumor imaging is usually enhanced by the conjugation of NPs with specific antibodies. Other moieties used in specific targeting include folic acid [59–61], peptides [62, 63] and cell ligands [64].
Engineered Polymers in Theranostic
Conventional cancer chemotherapy presents relevant limitations associated with the non-selectivity of cytotoxic drugs, their narrow therapeutic indices and limited cellular penetration. Furthermore, during the anticancer treatment, the real-time evaluation of the therapeutic efficacy is of considerable importance in the aim to evaluate patient response.
A possible interdisciplinary approach to overcome these drawbacks is the development of innovative therapeutic strategies involving the use of tumor-targeted nanodevices able to promote specific drug accumulation at the pathological site, but also able to act as diagnostic molecular imaging agents. In this context lies Theranostics also called theragnostics, which is an innovative and emerging treatment strategy that combines disease diagnosis with therapy and describes any material for this kind of applications. Theranostic approaches can involve the coupling of imaging with several types of therapy leading to imaging-guided drug delivery, but also image-guided gene delivery, photodynamic therapy, hyperthermia and radiation therapy [65]. Theranostic agents are characterized by the presence of both diagnostic and therapeutic functions within a single system that enables both diagnosis and targeted therapy at the same time, but also the monitoring of the therapeutic response to the treatment increasing the drug efficacy and safety.
In the last decades, the development of theranostic nanoparticles has received considerable attention due to their ability to be selectively delivered to tumors by passive or active targeting [66]. These TNPs, indeed, are designed for both cancer imaging (diagnosis) and treatment (i.e. chemotherapy or gene therapy).
A specific type of TNPs is the “activation” theranostic nanoparticles which are monitored for their kinetics in tumors. When imaging shows maximum nanoparticle accumulation in the tumor site, a physical source is applied in order to allow the burst release of the anticancer agent which is delivered rapidly and locally [1].
Different types of materials are used in the aim to prepare theranostic agents and, among them, imaging ones include photoluminescent mostly fuorescent groups, quantum dots [67], magnetic compounds and contrast agents for magnetic resonance imaging [68], while common therapeutic approaches are drug delivery [69], gene delivery [70], photodynamic therapy [71], hyperthermia and radiation therapy [71].
In this context, the present book chapter aims to focus the attention on polymer–based materials for theranostics.
A drug delivery system is defined as a device that enables the introduction of a therapeutic agent in the body and improves its efficacy and safety, with a reduction of the side effects, by controlling the rate, the time and the place of release. Polymeric nanoparticles are widely employed as effective DDS due to their ease of processing and the possibility to control their chemical and physical properties via molecular synthesis. Furthermore, the ability of nano-sized particles to preferentially accumulate in tumor tissues provides a platform for improved tumor diagnostics [50]. Therefore, by combining targeted delivery with advanced imaging technologies, it is possible to develop new polymer-based systems able to diagnose and treat cancer at the same time.
A polymer-based material for theranostics should consist of four main components (Fig. 10.2) [66]:
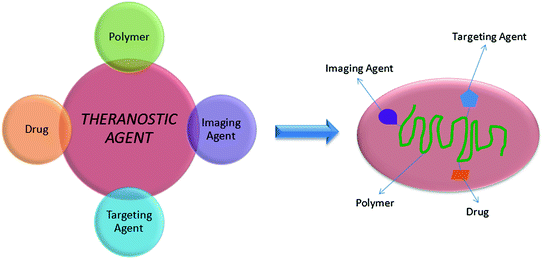
the polymeric component, which offers stability, biocompatibility, solubility and biodegradability;
the imaging component, such as quantum dots, magnetic nanoparticles or contrast agent;
the therapeutic component, that carries a drug, a gene or provokes therapy for example a photosensitiser that is used for photodynamic therapy;
the specific targeting agent, usually an antibody or peptide.
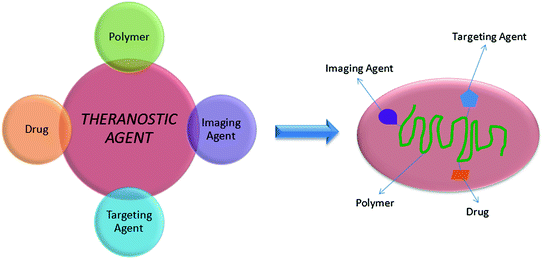
Fig. 10.2
Schematic representation of a theranostic agent and its main components
Image-guided drug delivery allows to monitor the biodistribution of the drug at real time and at the target site, quantify the drug release and evaluate the drug efficacy [69] while in image-guided gene therapy the incorporation of an imaging agent or labelled polymer or gene on the polyplex offers the ability to monitor the complex through the transfection/gene delivery procedure.
Polymer-Drug Conjugates
Polymer-drug conjugates offer several advantages such as increased circulations times, decreased toxicity, passive tumor targeting and incorporation of different functionalities including active targeting moieties or contrast agents [72–74].
N-(2-hydroxypropyl)methacrylamide is widely employed to prepare polymer-drug conjugates due to its non-toxicity, non-immunogenicity and stability in systemic circulation [75, 76] and several contrast agents have been associated with HPMA copolymer conjugates for in vitro and in vivo molecular imaging. Two different approaches are employed in order to functionalize HPMA copolymers with diagnostic and therapeutic moieties including copolymerization and chemical conjugation. The first one may be the preferred conjugation method due to the flexibility to conjugate single or multimodal diagnostic agents and chemotherapeutic drugs, while the other one allows the direct conjugation of the contrast agents and therapeutics to the copolymer.
Paramagnetically labeled HPMA copolymer conjugates were synthesized by free radical copolymerization of HPMA with monomers containing a chelating ligand, followed by complexation with Gd(OAc)3 [77], in the aim to study a non-invasive method of using contrast enhanced MRI to visualize the real-time pharmacokinetics, biodistribution and tumor accumulation of copolymer conjugates in tumor-bearing mice. Contrast enhanced MRI resulted in a real-time, three-dimensional visualization of blood circulation, pharmacokinetics, biodistribution and tumor accumulation of the conjugates.
In another study, polymeric nanocarriers based on HPMA copolymer and conjugated with low molecular weight drugs were designed in order to improve their efficacy [78]. The diagnostic system consisted of self-quenched Cy5 (SQ-Cy5) as near-infrared fluorescence probe and the therapeutic system was based on the anticancer agent paclitaxel. HPMA copolymer-PTX/SQ-Cy5 systems enable site-specific release upon enzymatic degradation in cathepsin B-overexpressing breast cancer cells.
Dendrimers
Among polymeric architectures, dendrimers are a unique class of repeatedly branched polymeric macromolecules characterized by three main parts including a core, an inner shell and an outer shell. They are versatile polymeric particles which can be designed for variable functionality for each of these parts and to feature controlled properties such as solubility and thermal stability. Due to the presence of many chain-ends and, therefore, improved reactivity properties compared to traditional linear polymers [79], dendrimers could undergo several chemical modifications for particular applications including imaging modalities. Furthermore, their surface functionalities provide the selective coupling of imaging agents, targeting ligands and/or other components to increase tumour specificity [1]. This kind of materials has been widely studied both in cancer therapy and diagnosis for example for photodynamic therapy (activation therapies) [80] and hyperthermia therapies using gold nanoparticles [81].
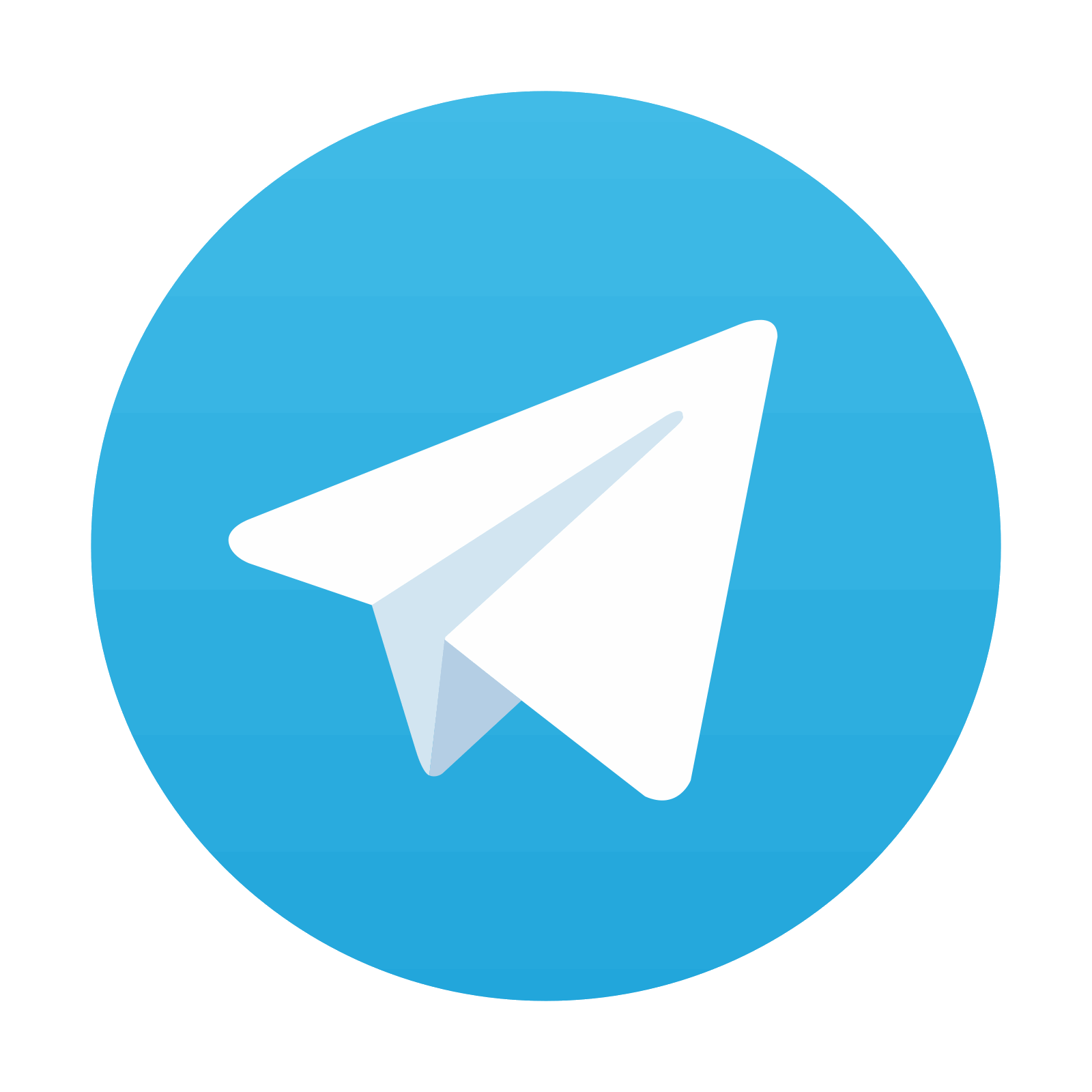
Stay updated, free articles. Join our Telegram channel
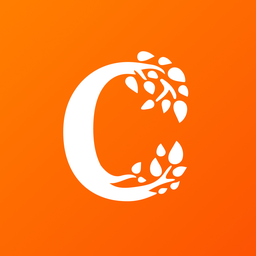
Full access? Get Clinical Tree
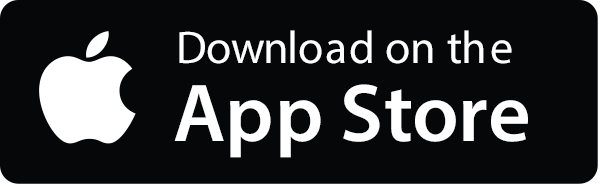
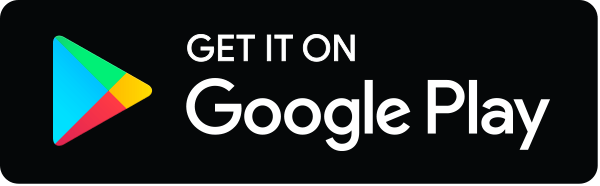