Polymer name
Manufacturer
Reason
Cuprophan®, Hemophan®
Membrana GmbH
Loss of market share, alleged lack of biocompatibility
Polycarbonate (PC)
Gambro
Only as flat sheet membrane available
Cellulose acetate (CA)
Cordis Dow
Loss of market share, manufacturing company dis-appeared from market
Membrane Polymers in the Market Place
The fate of the famous Cuprophan® dialysis membrane may serve as an example. Dialysis membranes, made from regenerated cellulose (e.g., Cuprophan®), used to be the golden standard for dialysis membranes for about four decades. Its manufacturers profited from a worldwide market share of more than 70 % in the 1970s. It could be shown, that the production capacity for Cuprophan® dialysis membranes paralleled the development of patient numbers in the 1970s. However in 2006 and about 35 years later, its main producer (Membrana GmbH, Germany) decided to cease the production of dialyzers with this membrane polymer. It can be argued that its alleged lack of blood compatibility and the availability of alternate biocompatible polymers provoked this development. A famous publication challenged this membrane polymer already in 1985 and thus, anticipated this situation by asking: “Cellulose membranes, time for a change?” [6].
Since then, only cellulosic dialysis membranes, made from derivatives of cellulose acetate, CDA and CTA, are commercially available. As a consequence, many scientific publications in which properties and outcomes of Cuprophan® application are compared are invalid today even if they are in favor of other membrane polymers. However, they are of importance and should not be neglected as they may serve for advising scientists on how to select polymers for developing new biomaterials.
For instance, the knowledge gained in these publications about adequate testing of membrane polymers, the effects of polymer composition to related clinical sequelae, etc., can be successfully used for the selection and the characterization of new polymers.
Polymers for New Therapies and the System’s Approach?
Unfortunately and despite many improvements in polymer behavior and performance, dialysis as a membrane-based procedure for blood purification is still an incomplete renal replacement therapy, as the mortality and morbidity of the affected patients remain high compared to the healthy population. To date, the following questions have not yet been answered satisfactorily:
1.
“Is it possible to improve patient mortality by either improved devices with optimized polymers, or by modified and more efficient treatment modes?”
2.
“Is it possible to improve patient survival through the introduction of completely new concepts, such as wearable artificial kidneys (WAKs) or through regenerative therapies?”
First successful attempts into this direction have been made with the introduction of convective therapies such as high-flux dialysis or hemodiafiltration (HDF). Increased pore sizes achieved with high-flux membranes allow for the removal of families of molecules and the possible high ultrafiltration rates improve removal by convective transport. The concept of a dialysis dose, based on the amount of a substitution fluid, called high-volume hemodiafiltration, turned out to be successful further [7, 8]. The ESHOL study published in 2013 [8] has proven a 30 % reduction of mortality in HD-patients given that this cohort has been treated by HDF with a substitution volume of more than 20 L. In a parallel arm of this clinical trial, patients were treated by normal high-flux dialysis. Obviously, treatment modes, such as high volume HDF combined with the application of high-flux membranes, has led to this advantage. As a consequence, polymers have to be suitable for this treatment mode and allow for an adequate ultrafiltration (water permeability), hydrophilicity and porosity in case of membranes.
Finally, it’s important to note, that a “systems approach” is obligatory when comparing different polymers in medical application. The exclusive study of the properties and characteristics of individual polymers in nephrology is not sufficient. In fact, one should consider a series of mutual interactions, e.g., between different polymers in a single device, their behavior within the device during different therapies, polymer interactions with the actual medication and other bystander conditions, such as long term stability [9]. Figure 13.1 depicts the variety of polymers used for medical devices in dialysis, such as dialyzers and tubing.
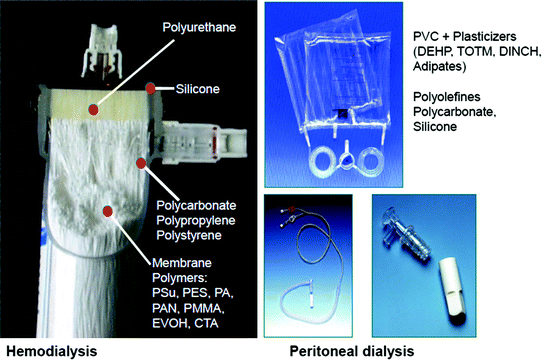
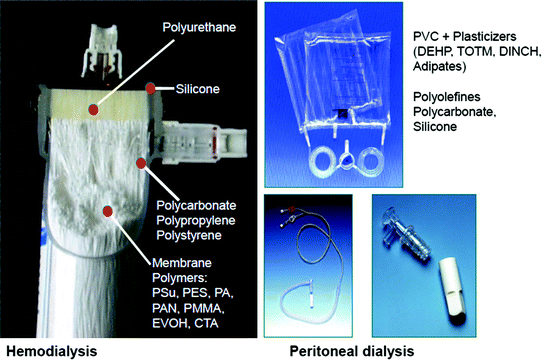
Fig. 13.1
Polymers used for medical devices for dialysis and peritoneal dialysis. Polyurethane (PUR) is applied as potting material for capillary membranes, silicone rings guarantee a leakage-free system, polycarbonate and polypropylene are used for casings, and a series of polymers are incorporated as membrane materials (PSu-polysulfone, PES-polyether-sulfone, PA-polyamide, PAN-polyacrylonitrile, PMMA-polymethylmethacrylate, CTA-cellulose-tri-acetate). PVC-polyvinylchloride as a biomaterial for tubing needs plasticizers for its flexibility, such as DEHP/DOP-di-ethyl-hexylphthalate/di-octyl-phthalate, TOTM-tri-octyl trimellitate, DINCH-di-isononyl-cyclohexane
This paper will discuss properties, requirements for use and characteristics related to possible clinical outcomes of polymers that are used in dialysis therapy.
Polymers for Dialyzers and Dialysis Membranes
For some people, it’s unbelievable and may sound like a surprise: 900 different types of dialyzers are commercially available worldwide in 2014. They differ in geometric design, membrane polymer and surface area, capillary geometry and undulation and can further be distinguished by their sterilization technique, steam, ethylene oxide gas, γ-rays, ß-rays, and E-beam.
The following questions may come up and bother clinic administrators and nephrologists:
Is there a real need for so many different types of polymers and filters?
Are so many filters desired by the nephrological community based on the specific healthcare requirements of dialysis therapies?
Is this market situation artificial and only stimulated by the international competition of dialyzer manufacturers?
Obviously, there is a need for so many different dialysis filters. The cohorts of dialysis patients vary in age, gender, body weight, blood volume, comorbid conditions, drug administration, predisposition to allergies and other physiological bystander conditions. Dialysis as a therapy for chronically ill patients tends to become more and more a practical model for personalized dialysis treatment regimen with respect to all patients. Thus, such a diversity of medical devices that is unprecedented in other realms of medical device technology, is needed.
Polymers for Dialyzer Housing
Recent years have seen a mayor change in the geometry of dialyzer housing and the corresponding membranes. For many years, Kiil dialyzers have been the work-horse of dialysis therapy. Here, the patient’s blood was circulated in a parallel flow mode through four cellophane channels made up of eight cellophane membranes. On the outside of the cellophane sheets dialysis fluid circulated at reduced hydrostatic pressure in the grooves of plastic boards [10]. The dimensions of the plastic boards were 970 × 340 × 20 mm and made of an epoxy resin compound. In fact, this size and the long lasting preparation time limited its use to a small number of patients. Improvements have been made with the introduction of the parallel plate dialyzers from Gambro/Hospal, fabricated with a polycarbonate housing and containing either a flat sheet Cuprophan or a PAN-membrane. Flat sheet dialyzers never dominated the market, because the mayor breakthrough came with the development of capillary-membrane dialyzers in 1968 [11]. Richard Steward, the first author on the first publication on capillary membranes spun his capillary membranes from saponified cellulose acetate polymers [11]. The concept of this device facilitated practical dialysis therapy and enabled manufacturers to produce filters in large quantities. There is certainly no doubt that the development of capillary dialyzers was the reason for the expansion of patient numbers worldwide. Figure 13.2 depicts the scheme of a modern capillary dialyzer.
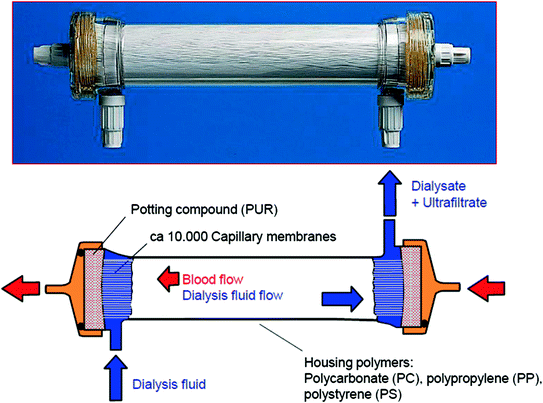
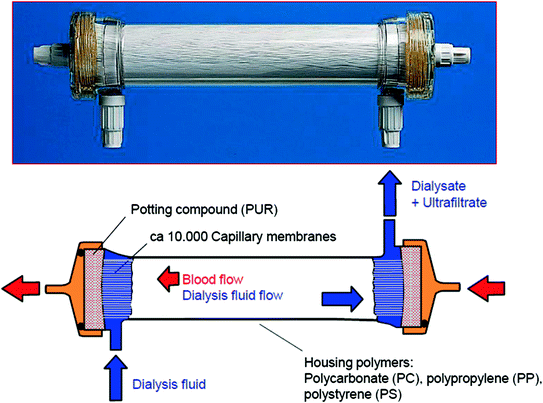
Fig. 13.2
The standardly used “artificial kidney” is a dialyzer that contains more than 10,000 capillary membranes. Blood is guided through these hollow fibers whereas the dialysis fluid is perfused in an opposite direction. Due to size, reproducibility and perfection of engineering features this type of dialyzer now dominates the dialysis market, mostly as a single-use device, since many years. The annual production capacity for hollow fibers in 2013 made from different polymers is about 450,000,000 km. This figure is equivalent to three-times the distance between the Earth and the sun
Standard dialyzer housings are made from polycarbonate. Its transparency and easy handling for labelling and sterilization is highly welcome by physicians and manufacturers. During the last decade, new developments have led to the introduction of housings made from two other polymers. Polypropylene (PP) was introduced for its polysulfone Fx-class by Fresenius Medical Care [12], and for the Sureflux-dialyzers provided by Nipro Medical Corporation, Japan. Polystyrene as housing material is available for PMMA dialyzers by Toray Industries, Japan.
Dialyzer cages made of polypropylene (PP) are not transparent, they appear opaque. However, they offer advantages that are appreciated by both, manufacturers and physicians. Medical grade PP is available at a cheaper price than medical grade polycarbonate which adds to lower manufacturing cost. In contrast to polycarbonate, PP does not release bis-phenol A (BPA). BPA is termed an exogenous hormone or endocrine disruptor because it interferes with the estrogen receptor at cell surfaces similar to the plasticizer di-ethyl-hexyl phthalate (DEHP). As a consequence of this interaction, BPA is made responsible for some adverse clinical effects, such as diabetes mellitus type II [13], impact on gene transcription [14], modulation of VEGF secretion [15]. Reports on dialysis patients describe its high protein-binding and thus explain its presence in the blood of dialysis patients in high amounts [16].
Compared to polycarbonate, however, handling of polypropylene in terms of labelling and sterilization needs special knowhow. It’s use as housing for medical devices is thus limited to engineering plastics experts.
Polymers for Potting of Capillary Membranes
Hollow fiber membranes must be fixed at both ends of a capillary dialyzer with a potting compound. Polyurethane has turned out to be the most suitable biomaterial for this purpose.
Polyurethanes (PUR or PU) are traditionally and most commonly formed by reacting a di- or poly-isocyanate with a polyol in a polyaddition reaction. While most polyurethanes are thermo-setting polymers that do not melt when heated, thermoplastic polyurethanes are also available.
Unfortunately, isocyanates are the most frequent cause of occupational asthma in industrialized countries. Exposure to isocyanates may result in the formation of specific IgE antibodies [17, 18]. There are also reports in the literature, that 4,4′-Methylene-di-aniline (MDA) can be released from aromatic polyurethanes after gamma-irradiation or steam sterilization [17, 19] suggesting the advantage of aliphatic polyurethanes in contrast to its aromatic derivatives.
Finally, polyurethane polymers used as potting material in dialyzers are known for effectively binding and adsorbing the sterilization gas ethylene oxide (ETO). Due to the very slow release kinetics of ETO, a dialysis patient may be continuously exposed to the gas and IgE-antibodies against an albumin-ETO conjugate are found in dialysis patients and severe allergic reactions could be observed in hypersensitive patients [18]. A rigorous rinsing of the dialyzer prior to its clinical use allowed for avoiding this “first-use syndrome”. Dialyzer manufacturers meanwhile have improved the quality of dialyzers by reducing the PUR potting mass to its minimum necessary amount.
Polymers for Dialysis Membranes
The selection of the polymer material for the production of dialysis membranes has significant implications on the quality of hemodialysis therapy. Only a limited number of polymers are suitable for the spinning and extrusion process involved in the manufacture of capillary membranes. The selection was originally based on experiences from textile fiber production. Further, biochemical and physical properties that may result from the membrane formation process, determine the selection of a polymer. The ideal polymer family should allow the production of biocompatible dialysis membranes and possess a stable physical stability. The latter guarantees an easy production process and sterilization without problems.
The majority of patients with kidney disease are currently treated with dialyzers containing synthetic membranes with high-flux properties [20]. Figure 13.3 depicts changes in market shares for membrane flux and polymer type from the year 2000 to 2013 [21]. The figure shows that membrane polymers have evolved as part of a routine therapy, essentially in line with clinical observations and constantly changing clinical requirements [20].
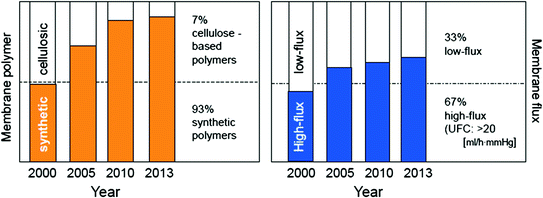
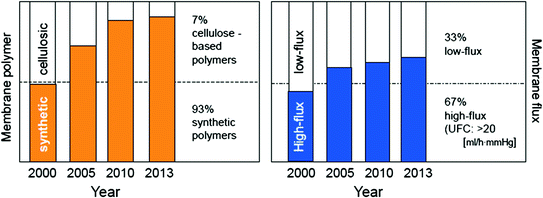
Fig. 13.3
Worldwide changes of dialysis membrane properties observed between the years 2000 and 2013. Cellulose-based membranes have lost their importance and market share continuously. They are replaced by synthetic polymers as membrane materials. In addition “flux” has become an issue either. High-flux membranes, characterized by their ultrafiltration coefficient to be UFC => 20 [ml/h·mmHg] are now used in more than two thirds of all dialysis centers
Eighteen different membrane polymers can be attributed to four synthetic and to one cellulosic membrane family. They will be described shortly in the following and characterized by features that outline their specific individual advantages or disadvantages. Table 13.2 depicts an actualized list of polymers used for dialysis membranes and the appropriate manufacturers of dialyzers. Their characteristics will be discussed below.
Table 13.2
Dialysis membranes listed according to their polymers and dialyzer manufacturers
Type of polymer (polymer groups) | Membrane manufacturer (in alphabetic sequence, *exclusive membrane producer) | Dialyser manufacturer (if different from membrane manufacturer) | Dialyser types (examples) |
---|---|---|---|
Synthetic dialysis membranes (polymer groups) | |||
Polysulfone (PSu) membranes | |||
Asahi Polysulfone (APS™) | Asahi Kasei Medical Co., Ltd., Tokyo, Japan | APS-650 Leoceed-16 N | |
REXBRANE™ | REXEED™-13A | ||
VitabranE™ | ViE-A, ViE | ||
α Polysulfon | BBraun Melsungen AG, Melsungen, Germany | Diacap®HI PS 12 | |
α Polysulfon+ | Diacap®HIFlo 23 | ||
Amembris | Xevonta Hi 12 | ||
Fresenius Polysulfone® | Fresenius Medical Care AG, | Hemoflow F60S | |
Helixone® | FXclassix60 | ||
Helixone®plus | Bad Homburg, Germany | FXCordiax60 | |
Fibron Polysulfone | Fibron AG*, Teterow, Germany | Allmed Medical GmbH, Pulsnitz, Germany | Polypure® 16H |
Haidylena, Giza, Egypt | Platinum H3 | ||
Minntech Polysulfone | MEDIVATORS Inc. (previously Minntech), Minneapolis, USA | Renaflo® II | |
Toraysulfone® | Toray Medical Co., Ltd., Chiba, Japan | TS-1.3S | |
Poly(aryl)ethersulfone (PAES) membranes | |||
Polyamix™ | Gambro Dialysatoren GmbH, Hechingen | Polyflux®140H | |
Poracton™ | Revaclear™ | ||
HCO—membrane | Germany | Theralite™ | |
DIAPES® | Membrana GmbH*, Wuppertal, Germany | Allmed Medical GmbH, Pulsnitz, Germany | Ruby 160 |
Bellco S.r.l., Mirandola, Italy | BLS 812G | ||
Haidylena, Giza, Egypt | HPH 160 S | ||
Medica S.p.A., Medolla, Italy | L3 | ||
Meditechlab, Thiais, France | MF 7 | ||
Nipro Corp., Osaka, Japan | SureLyzer® PES-150DH | ||
Purema® | Membrana GmbH*, Wuppertal, Germany | Allmed Medical GmbH, Pulsnitz, Germany | Biorema 16H |
Bain Medical Equipment Co., Ltd, Guangzhou, China | DORA B-16H | ||
Baxter International Inc., Deerfield, USA | Xenium 130LF | ||
Bellco S.r.l., Mirandola, Italy | Phylter™15G | ||
Etropal JSC, Etropole, Bulgaria | PH 160 | ||
Haidylena, Giza, Egypt | Biopure 160 HF | ||
Medica S.p.A., Medolla, Italy | Smartflux HFP 150 | ||
Meditechlab, Thiais, France | MP 60 | ||
MTP Medical Technologies Gesellschaft mbH, Pirna, Germany | VitaPES® 150 HF | ||
Nephros Inc., River Edge, USA | Olpur™ | ||
Nipro Corp., Osaka, Japan | PureFlux®-130H, | ||
NxStage GmbH & Co. KG, Göttingen, Germany | Filter in System One®-Cassette | ||
Polyester-Polymer Alloy (PEPA®) | Nikkiso Co., Ltd., Tokyo, Japan | FLX-15GW | |
Polynephron™ | Nipro Corp., Osaka, Japan | Nipro Corp., Osaka, Japan | ELISIO™-13H |
Baxter International Inc., Deerfield, USA | Xenium XPH130 | ||
Polyacrylonitrile (PAN) membranes | |||
AN69, AN69®ST | Gambro Hospal | Gambro Hospal/Baxter Healthcare | Nephral®ST |
HeprAN™ | Meyzieu, France | Evodial®1.3 | |
Other synthetic dialysis membranes | |||
Ethylene vinyl alcohol copolymer (EVAL™, EVOH) | Asahi Kasei Medical Co., Ltd., Tokyo, Japan | KF-201-1.3C | |
Polymethylmethacrylate (PMMA) | Toray Medical Co., Ltd., Chiba, Japan | Filtryzer® BG-1.3U | |
Cellulose-tri-acetate (CTA) membranes | |||
Cellulose-tri-acetate (CTA) | Nipro Corp., Osaka, Japan | Nipro Corp., Osaka, Japan | SUREFLUX™-13L |
Baxter International Inc., Deerfield, USA | TRICEA 150G |
Membrane Families, Their Main Features and Specific Properties?
Polymers for the manufacturing of dialysis membranes can be grouped as families and are described in the following.
Group 1, Polysulfone-family Members: Polysulfone (PSu, Fresenius Medical Care, Germany), Polyethersulfone (PES, Membrana, Nipro Medical Corporation, Japan), Polyester polymer alloy (PEPA, Nikkiso, Japan), Blends from Polyamide/Polysulfone (PA/PSu, Gambro, Sweden), as well as blends made of PES/PVP. Recent research on membranes with antioxidant features have led to the production of a polysulfone membrane with immobilized Vitamin E (PSu/Vit E, ASAHI, Japan).
Membranes from polysulfone dominate the dialysis market since many years. Today, 93 % of all synthetic membranes stem from the parent polyarylethersulfone family of which 71 % are from polysulfone (PSu) and 22 % from polyethersulfone (PES). Polysulfone belongs to a family of thermoplastic polymers which are known for their thermal and chemical stability. They contain the subunits “aryl-SO2-aryl” and “benzyl-SO2-benzyl” (Fig. 13.4) of which the polysulfone group provides the name of the polymer.
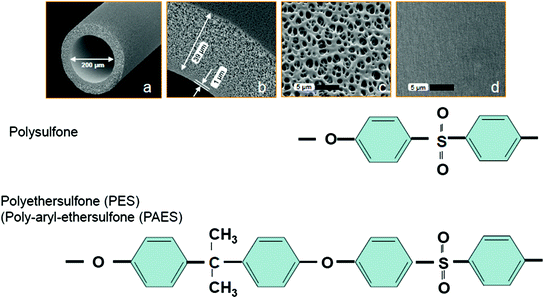
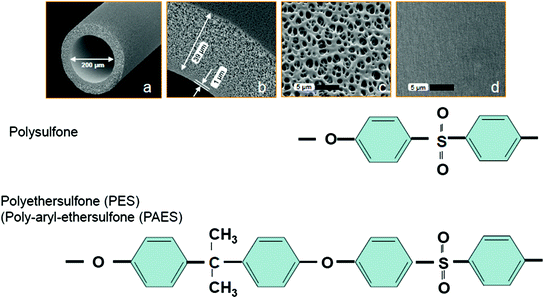
Fig. 13.4
Chemical structure and characteristics of polysulfone (PSu) polymers used for dialysis membranes. Its specific chemical characteristics are shown in the panel above. PSu- membrane dimensions are depicted in the centered panel: a capillary cross section, inner diameter 200 µm, b cross section of the membrane wall; the membrane is only 1 µm thick and backed by a rather open support structure of 39 µm that maintains mechanical stability, c view on the outer membrane surface area, showing its high porosity, d view on the rather smooth inner membrane surface area where pore sizes are between 1 and 3 nm. The lower panel shows the detailed molecular structure of polysulfone (PSu) and polyethersulfone (PES)
What Is the Difference Between Polysulfone (PSu) and Polyarylethersulfone (PES/PAES)?
Polysulfone, polyethersulfone and polyarylethersulfon are used as synonyms by many scientists due to their great similarity in performance and chemical stability. Generally spoken, polysulfones (PSu) are a group of polymers that contain sulfone- and alkyl- or aryl- (e.g., arylether-) groups. According to the chemical nomenclature, only those polymers belong to the polysulfone family, that contain an additional isopropylidene group. Polymers without the isopropy-lidene group are called polyarylethersulfones (PAES) or in short polyethersulfones (PES) (Fig. 13.4).
Modification of PSu Membranes
The polysulfone polymer, as such, is a hydrophobic polymer and must be rendered hydrophilic at least in part. This is generally achieved through blending PSu with polyvinylpyrrolidone (PVP). A PSu/PVP blend allows for both, an efficient ultrafiltration profile of the final membrane, and the formation of a domain-like surface structure with hydrophilic and lipophilic spots at the blood contacting surface. Already in 1983, Streicher and Schneider reported on their clinical experiments in dialysis with the first PSu membrane in its high-flux version. To their surprise, they were able to observe a high sieving coefficient (SC = 0.7) and an efficient removal of ß2-microglobulin and compared its performance with the glomerular basement membrane [22]. This was long before the removal capacity of ß2-microglobulin was considered of paramount importance for membrane efficiency in later years. The success of this membrane polymer can be further attributed to appropriate and efficient solute and fluid removal capabilities and to a pronounced intrinsic blood compatibility [23].
It is possible to affect blood compatibility and biocompatibility of so called non-physiological interfaces by chemical modifications of the polymer contact zone. The contact zone between blood and the membrane polymer represents such a non-physiological interface [24]. Blood compatibility of artificial surfaces is primarily determined by protein adsorption and protein adsorption depends on surface properties of the membrane. A general scheme of how surface reactivities are influenced by the chemical composition of a membrane is depicted in Fig. 13.5.
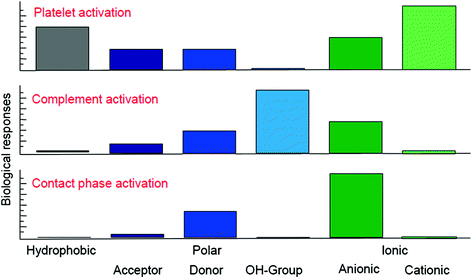
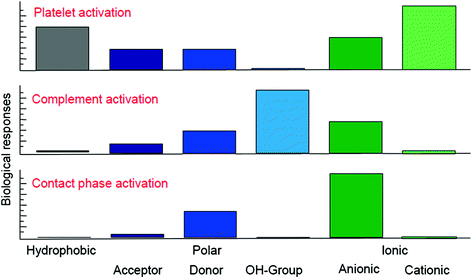
Fig. 13.5
Overall biological response as a function of surface properties. Inert surfaces for the anticoagulation system are hydrophobic, cationic charged and OH-group bearing. Inert systems for complement are also hydrophobic and cationic (modified from [24])
As a consequence, none of the surfaces with single-characteristics properties satisfies biocompatibility sufficiently. Domain-like surface structures are essential in order to achieve optimized blood compatibility (see above). Poylsulfone/polyvinylpyrrolidone blends (PSu/PVP) offer such domain-like structures with the well-known biocompatibility features.
PSu Membranes and Endotoxin Binding Capacity
A further major advantage of PSu-membranes relates to their adsorptive capacity for bacterial endo-toxins. Dialysis therapies with high-flux membranes expose the patients to large volumes of dialysis fluid, e.g., during high-volume hemodiafiltration [7, 8]. Should these fluids be contaminated with endotoxins that derive from the outer cell wall of Gram-negative bacteria during cell growth or lysis, there is a risk of these substances entering the patient’s bloodstream [25, 26]. Endotoxins activate a number of physiological pathways, triggering the generation of potent pro-inflammatory substances, such as cytokines (IL-1, IL-6, TNF, etc.) and growth factors (e.g., VEGF) [26]. Subsequent inflammation, the underlying mechanism for most related disease states including chronic kidney failure, is widely recognized to be a major risk factor for cardiovascular disease-related mortality in dialysis patients.
Depending on their chemical constitution and microstructures, polysulfone membranes are able to prevent entry of endotoxins into the patient’s blood stream [27–29, Fig. 13.6]. However, polysulfone and polysulfone membranes are not all alike.
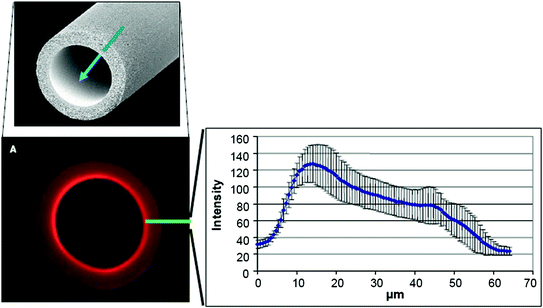
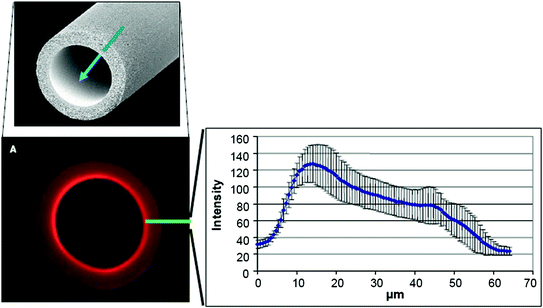
Fig. 13.6
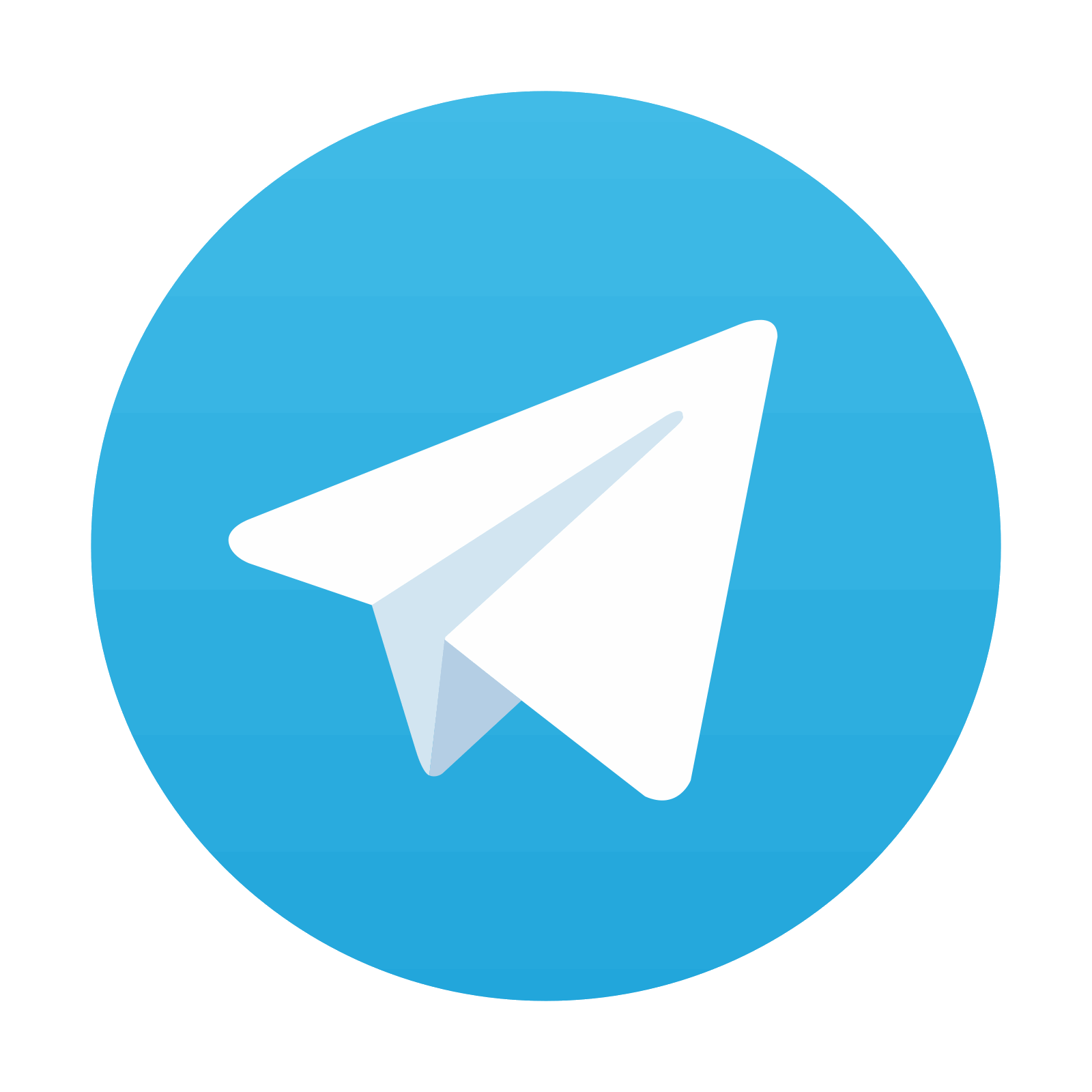
Endotoxin adsorption at the inner layer of a membrane made of a polysulfone/poylvinylpyrrolidone blend. This adsorption capacity prevents endotoxins possibly originating from contaminated dialysis fluid to enter the patient’s blood stream
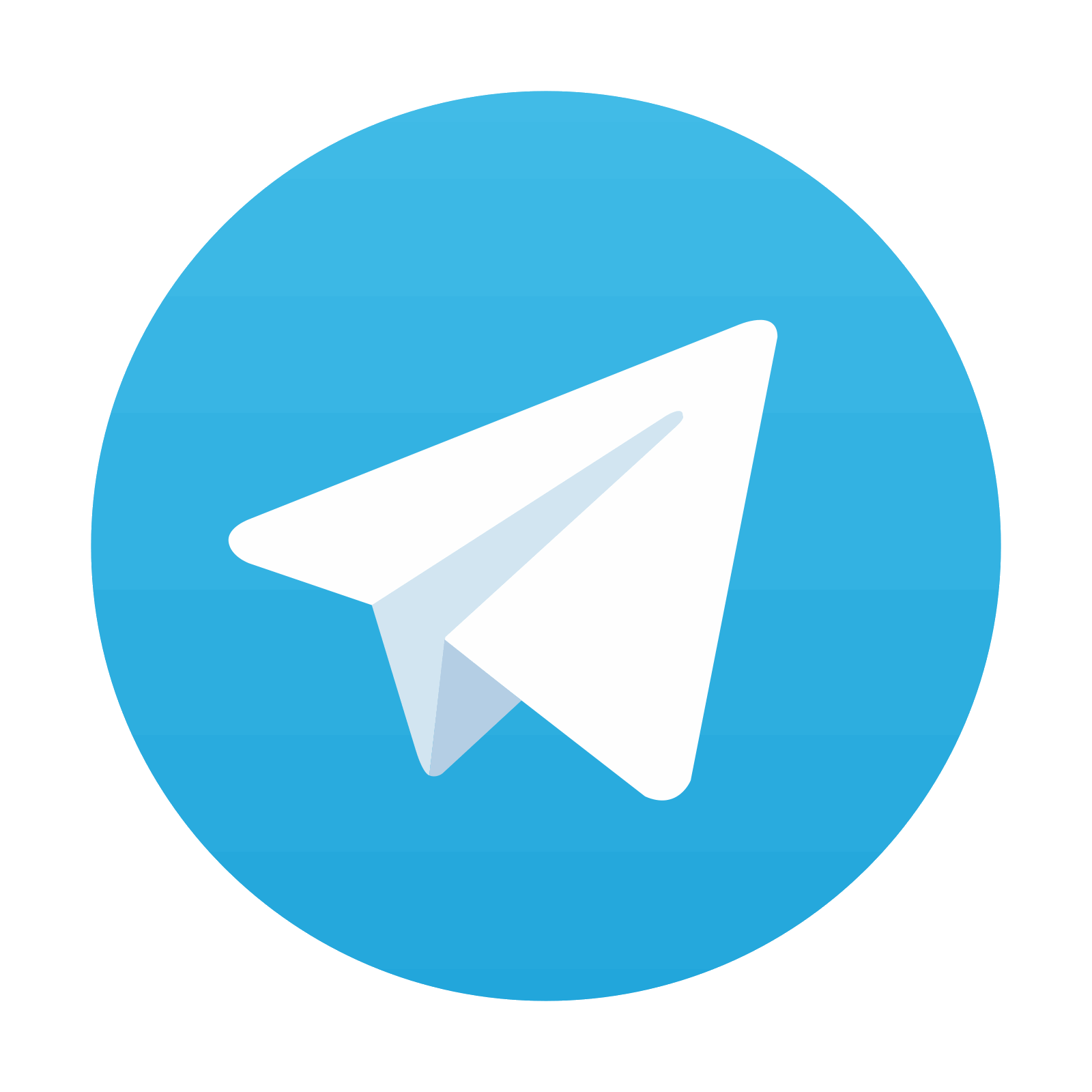
Stay updated, free articles. Join our Telegram channel
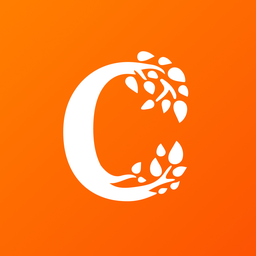
Full access? Get Clinical Tree
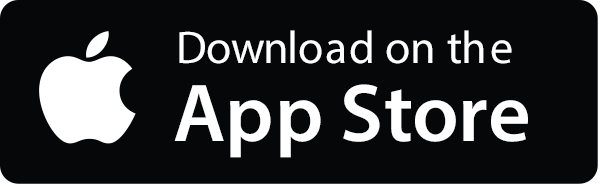
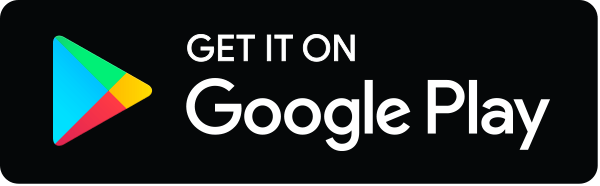
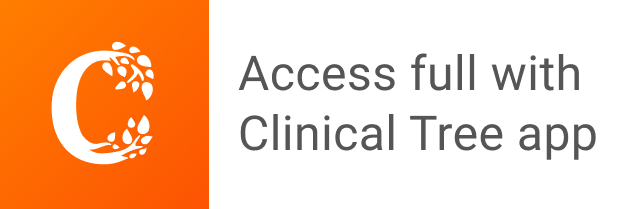