Fig. 11.1
Medicines can be designed: (i) to exert a local effect on the application site by means of drug release and penetration in surrounding cells (a), (ii) to exert a systemic effect by releasing the drug in a place suitable for drug absorption (b) and/or biodistribution (c), (iii) or to target the drug to profound specific tissues or cells via direct transport of the drug (inside nanocarriers) through the blood stream towards the target (d). In either case, the drug has to be released from the dosage form to get access to the pharmacological target and exert the therapeutic effect, but the release occurs in different sites and at different rates
When the medicine is administered through a non-parenteral route, the drug has to be transferred from the dosage form to the neighbor biological environment. Dissolved drug molecules can either accumulate in the tissue in contact with the dosage form (Fig. 11.1a; e.g., skin, ophthalmic or bronchial application) for local treatment, or can pass through the membranes and enter into the bloodstream (Fig. 11.1b), from where the drug will be distributed throughout the body, reaching the site of action (systemic effect) and the elimination organs. Intravenous solutions allow direct incorporation of the drug to the blood stream (Fig. 11.1c). Parenteral route offers also the possibility of administering colloidal (nanometric) carriers with the drug encapsulated for specific delivery to specific tissues or cells (targeting) (Fig. 11.1d).
Except for target delivery systems, drug release and passage through biological membranes are steps that occur sequentially. As a consequence, the slower process determines the overall rate of transfer of drug from the dosage form to the blood circulation; that is, the slower process is the limiting step for drug absorption. If both drug absorption and clearance are rapid, repeated administrations at short time intervals may be required to maintain effective plasma levels. The possibility of modulating the rate of absorption of a drug by applying technological means able to adjust the release from the dosage form is the basis of the modified release dosage forms. This group comprises prolonged (sustained)-release, delayed-release and pulsate-release forms (Table 11.1).
Table 11.1
Classification of drug dosage forms according to drug release profile as stated in the USP 37–NF 32 (2014)
Dosage form | Drug release pattern | Performance |
---|---|---|
Conventional | Immediate release | Release depends on the physico-chemical properties of the drug |
Modified release | Prolonged | Release occurs at slower rate than from conventional dosage form |
Delayed | Release does not occur until a certain lag time or a certain stimulus triggers it | |
Pulsate | Sequential release pulses of one or more therapeutic substances |
Prolonged release dosage forms, also known as rate-programmed release systems, pursue simplification of dosing regimens, by spacing the intervals between successive administrations of doses, and attenuation of peaks in drug levels [5]. The second generation of controlled release systems, called activation-modulated ones, appeared to regulate not only the rate, but also the site at which drug release should occur. The first devices were developed for site-specific release of orally administered medicines, but rapidly adapted to other routes. In these systems, drug release is activated by physical (swelling), chemical (e.g., pH) or biochemical (e.g., enzymatic degradation) stimuli. Common examples of oral delayed-release formulations are the enteric systems for release in the intestine of irritant or labile drugs, and the time-retard systems for fitting of drug release to circadian rhythm of pathological processes. Some pulsate-release formulations have been developed for sequential release of one or various therapeutic substances by combining particles that degrade at different rates after activation; parenteral administration of pulsate-release systems has been successfully applied for vaccination. Most modified-drug release devices commercialized belong to the two first generations. The third generation of controlled release systems pursues the feedback-regulation of drug release, by testing the patient condition, especially illness evolution. These new systems present the singularity of integrating components that play an active role in the therapeutic treatment [6].
Polymer Excipients
As introduced above, the roles that a given dosage form is expected to play are very varied, but can be gathered into three large groups: (i) facile drug dosing and patient acceptability; (ii) enhanced safety of the treatment and reproducibility of the therapeutic response; and (iii) improved drug efficacy (Table 11.2). The dosage form has to provide the adequate physical properties to facilitate the administration, but also have to correct/modulate physicochemical and biopharmaceutical properties of the drug, such as solubility or permeability, which are critical for the effectiveness of treatment. Poor aqueous solubility is a common problem among new chemical entities, because the current drug discovery methods favor the selection of highly hydrophobic molecules. In other cases, hydrophilicity and large molecular weight hinder the pass through biological membranes. Current diversity of requirements regarding spatio-temporal regulation of drug release also make design and development of drug dosage forms a highly challenging task. To ensure the quality of medicines, the dosage form has to contribute to the chemical and physical stability of the drug, both in the course of the manufacturing process and during storage.
Table 11.2
Main functions of the drug dosage forms
Category | Performance |
---|---|
Facile drug dosing and patient acceptability | Adequate physical format for administration |
Simple and rational dosing | |
Correction of unpleasant organoleptic properties | |
Enhanced safety of the treatment and reproducibility of the therapeutic response | Improved physical and chemical stability of the drug |
Protection against microbial contamination | |
Minimization of toxicity and untoward effects | |
Improved drug efficacy | Modulation of drug solubility, release rate and permeability |
Enhanced bioavailability | |
Modulation of drug biodistribution | |
Easy adherence to the treatment |
The diversity of roles that dosage forms are called to play is only possible thanks to strong efforts in the finding/synthesis of suitable excipients able to face up to the increasing complexity of production processes and increasingly stringent quality requirements. The United States Pharmacopeia (USP37/NF32, 2014) classifies the excipients in more than 40 functional categories according to their most common application in the formulation of pharmaceuticals. Such large list of functions can be reorganized in main four categories:
Processability, i.e., they facilitate the manufacture of the dosage form;
Stabilization, to overcome problems related to the stability of the drug or the dosage form;
Correction of organoleptic properties; and
Modulation of drug bioavailability (altering solubility, permeability or drug release rate) or the access (targeting) to specific tissues or cell structures.
As material science progresses, novel and everyday more sophisticated materials are being evaluated as excipients for drug dosage forms. It should be noticed that, among other materials, polymers occupy a relevant position as versatile excipients able to perform one or more functions simultaneously. Multifunctional excipients are particularly attractive because they simplify development and manufacturing processes and reduce costs. Currently, natural, semi-synthetic and synthetic polymers are common excipients (Fig. 11.2). Natural polymers, mainly polysaccharides and protein derivatives, have been largely used for many years to cover the demands of classical dosage forms (tablets, capsules, gels) and are still widely used today because the good biocompatibility of most of them, biodegradability in the body or in the environment, and obtaining from renewable sources. Improvements in the extraction and characterization methods make natural polymers reliable materials for quality standards. Moreover, some natural polymers perform as multifunctional or can be easily derivatized to modulate their solubility and biodegradability patterns or to endow them with responsiveness to certain stimuli. Synthetic polymers have the advantage of being prepared on demand to fit to specific requirements, setting a priori their functional groups and molecular weight. Outstanding examples of biodegradable and non-biodegradable polymers are poly(l,d-lactic-co-glycolic acid) (PLGA) and polyethylene glycol (PEG), respectively.
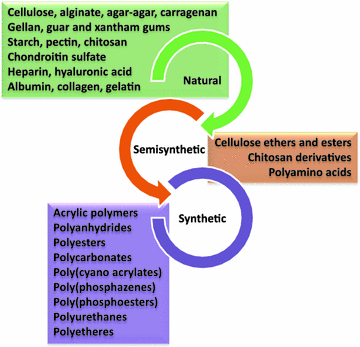
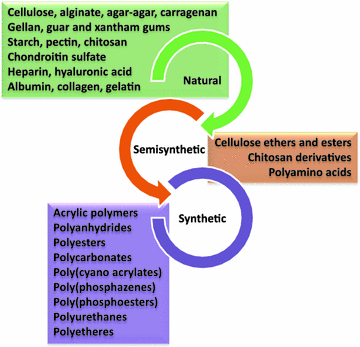
Fig. 11.2
Examples of natural, semi-synthetic and synthetic polymers used to prepare drug dosage forms
In the next sections, examples of polymer excipients suitable for specific functionalities are analyzed.
Polymers as Manufacture Aids and Sustained Release Formulations Components
Processing requirements are notably different depending on the physical state of the dosage form. Manufacture of dosage forms requires excipients that are able to overcome deficiencies of the drug regarding processing and contribute to rapid and reproducible production of manageable medicines. Hydrophilic polymers may also contribute to slow down drug release by increasing the viscosity of liquid formulations or by forming gel matrix networks when solid formulations become wet. In contrast, hydrophobic polymers can help regulate drug release by means of hydrolytic or enzymatic degradation mechanisms.
Cellulose and Cellulose Derivatives
Cellulose is the main structural component of cell walls in higher plants and consists in linear, unbranched polysaccharide of β-1,4-linked d-glucose units. Cellulose chains form long crystalline microfibrils aligned with each other’s to provide structural support to the cell wall [7]. The strong structure of cellulose provides a high resistance against enzymatic reactions that degrade the chains. It is also insoluble in water and indigestible by the human gastrointestinal system. Modifications of cellulose structure leads to materials widely used in the pharmaceutical field to facilitate the manufacture of drugs:
Microcrystalline cellulose (MCC). It is obtained by treatment of cellulose with hydrochloric acid to partially depolymerise the structure. After purification by filtration and spray-drying, a free-flowing powder of small aggregates of cellulose fibers is obtained [8]. Polymer compactability can be modified by varying the degrees of polymerization and of crystallinity. It is commonly used as diluent in wet granulation and as filler in capsules. The most known commercial brands are Avicel® and Emcocel®.
Silicified MCC. Commercialized as Prosolv SMCC®, it is produced by co-processing MCC (98 %) with colloidal silicon dioxide (2 %) and commonly used as filler binder and glidant [9]. This excipient has better properties for direct compaction, such as flowability and packaging properties. In addition, silicified MCC has higher bulk density than does regular MCC and maintains compactation properties after wetting and drying.
Cellulose ethers. Derivatives more hydrophilic than cellulose and thus that can easily disperse in water are obtained via substitution of one or more hydroxyl groups of the d-glucose units with alkyl, hydroxyalkyl and carboxyalkyl molecules. Applications of cellulose derivatives depend on the degree of substitution because it determines the strength of the interaction with water. Non-ionic cellulose ethers, such as hydroxypropylcellulose (HPC) and hydroxypropyl methylcellulose (HPMC) are commonly used in tableting as binders and film-coating components. Gelling features of medium-high substituted cellulose ethers make them suitable to create gel barriers when tablets enter into contact with physiological fluids, which can sustain drug release. They are also suitable as thickener agents for the formulation of solutions and suspensions. Low substituted non-ionic cellulose ethers behave as disintegrants of tablets and pellets [10]. Co-processed excipients containing MCC, HPMC and crospovidone show excellent flowability, compressibility and mixing ability, and therefore notably improve manufacturability of solid dosage forms.
Starch
Starch is a storage carbohydrate consisting of glucose monomers organized as amylose and amylopectin chains. Starches are used in solid oral formulations as diluents, binders and disintegrants. Deficient flow properties of natural starches can be overcome by substitution with acetate groups, prepared by partial reaction of the hydroxyl groups of starch with acetic acid anhydride in an acetyl esterification reaction [8, 11]. Alternatively, flow properties can be improved via chemical and/or mechanical processing to break all or part of the starch granules (namely, the bonds between amylose and amylopectin molecules) in a process named pregelatinization. Partial pregelatinization renders the starch flowable, directly compressible, and suitable for preparing oral capsules. Both partial and fully pregelatinized can be used in wet granulation processes. Combination of pregelatinized starch with amylose and amylopectine leads to multifunctional excipients that are effective as binders, disintegrants, flow aids and lubricants [12]. Most known commercial brands are Starch 1500®, Sepistab ST200® and C*PharmGel®.
Natural Anionic Polysaccharides
Anionic polysaccharides extracted from seaweeds (alginate, agar, carrageenans; Fig. 11.3) and plant cell walls (pectin) and exudates (gum arabic) are largely used as thickening and gelling agents. Alginate is a linear polysaccharide composed of β(1-4)-d-mannosyluronic acid (M) and α(1-4)-l-gulosyluronic acid (G) blocks. The ratio and the distribution of the M and G blocks (Fig. 11.3) notably affect to the sensitiveness of alginate to pH and calcium ions because the different relative position of the carboxylic acid group in each block [13]. Alginate also offers a wide range of possibilities of derivatization to improve its performance [14]. Alginic acid and its sodium, calcium, ammonium and potassium salts have been shown suitable for oral and topical dosage forms, acting as diluent and disintegrant in solid forms and as thickener, emulsifier and taste-masking agent in liquid and semisolid. Alginic acid is particularly suitable for preparing microcapsules, pellets and microspheres that are easily cross-linked in the presence of calcium ions [15].
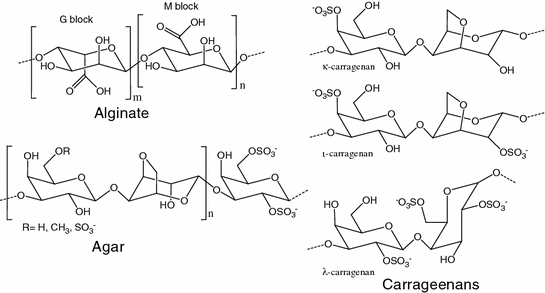
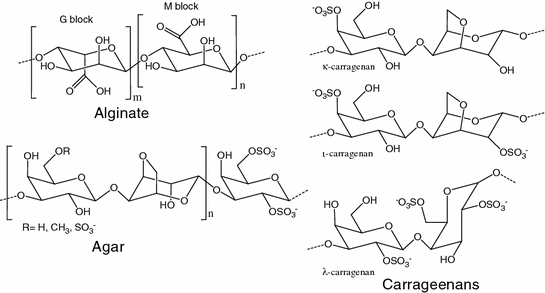
Fig. 11.3
Structure of some anionic polysaccharides used as pharmaceutical excipients
Carrageenans or carrageenins are hydrocolloids extracted from some red seaweeds. They mainly consists of linear β-d-galactose and 3,6-anhydro-α-d-galactose copolymers with a variable density in sulfated groups, which leads to three different families: kappa (κ, 1 sulfate group per dimer) which has a helical tertiary structure and strong gelling capability; iota (ι, 2 sulfate groups per dimer); and lambda (λ, 3 sulfate groups per dimer) which does not form gels (Fig. 11.3). Only κ-carrageenan hydrogels exhibit pH- and temperature-sensitiveness [16]. At low concentration, carrageenans are added to emulsions to increase the physical stability. They are also used as pelletizer agents for extrusion-spheronization, binder agents in tableting, and as components of hard and soft capsule shells.
Agar or agar-agar is obtained from the cell walls of some species of red algae or seaweeds and is widely employed as emulsifying and suspending agent, thickener, and tablet binder. Agar is a heterogeneous mixture of two unbranched polysaccharides: agaropectin and agarose, which share the same galactose-based backbone (Fig. 11.3). Agaropectin is heavily modified with acidic side-groups, such as sulphate and pyruvate, while agarose has neutral charge and possesses longer chains [17]. Thus, depending on the composition, agar are designed as neutral agarose, pyruvated agarose (with little sulfation) and sulfated galactan.
Polyvinylpyrrolidone
Polyvinylpyrrolidone (PVP), also known as povidone and commercialized as Kollidon® (Fig. 11.4), is a synthetic linear polymer widely used in pharmaceutical industry. It is highly soluble in water, but also in some organic solvents such as butanol. The thickening ability of PVP depends on its molecular weight, and several grades can be distinguished which are identified with a K-value (average molecular weight estimated from relative viscosity values). PVP is also used as adhesive and binding agent in tablets, and as taste masker in oral solutions and chewing tablets. Chemically and physically cross-linked insoluble PVP (crospovidone) has good flow properties and strong swelling capability, performing as disintegrant of tablets. Water-soluble copolymers of N-vinylpyrrolidone and vinylacetate (copovidone; Fig. 11.4) are used as binders and as film-forming agents of tablets, pellets and granules. Compared to PVP grades, copovidone has lower hygroscopicity, better dry binding properties, and higher plasticity [18].
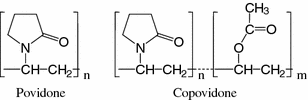
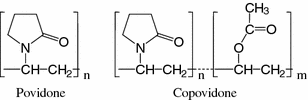
Fig. 11.4
Repeating units of povidone and copovidone
Acrylic Acid-Based Polymers
High molecular weight, crosslinked, acrylic acid-based polymers are known as Carbopol® and Noveon®. Carbopol® homopolymers consists of acrylic acid crosslinked with allyl sucrose or allylpentaerythritol. There exist also Carbopol® copolymers (with C10-C30 alkyl acrylate) and interpolymers (which contain a block copolymer of polyethylene glycol and a long chain alkyl acid ester). Noveon® polycarbophil is a polymer of acrylic acid crosslinked with divinyl glycol [19].
These polymers have microgel structure and behave as thickening agents in lotions, creams and gels, oral suspensions, and in transdermal gel reservoirs. They are also useful to communicate controlled release properties to solid dosage forms. In contact with the aqueous media, acrylic acid-based polymers form gel matrices that hinder drug diffusion more efficiently than other polymers. It should be noticed that due to the presence of carboxylic acid groups, acrylic acid-based polymers undergo relevant conformational changes as a function of pH and ionic strength. At pH above the pKa (5.5–6.5), the ionized polymer chains expand and can easily entangle with neighbor chains forming highly structured, viscoelastic gels.
Polyesters
Polyesters are biocompatible and biodegradable polymers including poly(lactic acid) (PLA), poly(glycolic acid) (PGA), poly(lactic-co-glycolic acid) (PLGA), and poly-ε-caprolactone (PCL). These biocompatible polymers are widely used to encapsulate drugs in micro/nanoparticles and in macroscopic implants [20, 21]. Poly(α-hydroxy) esters are not biodegraded by enzymes in the body, but undergo hydrolytic degradation via surface or bulk degradation pathways. Surface degradation occurs when the rate of hydrolytic chain scission and the production of oligomers and monomers which diffuse into the surroundings is faster than the rate of water intrusion into the polymer bulk. As a consequence, the formulation erodes over time without affecting the molecular weight of the polymer bulk. Bulk degradation occurs when water penetrates the entire polymer structure, causing hydrolysis throughout the entire polymer matrix and thus overall reduction in molecular weight. In the case of PLGA, if the generated carboxyl-containing species do not diffuse out the matrix, they can act as internal autocatalyzer that accelerates the bulk degradation compared to the surface. Due to these biodegradation patterns, PLGA formulations exhibit bi/triphasic release profiles: initial burst of drug close to the surface, slow release of the entrapped drug until the molecular weight of the polymer becomes lower than a critical threshold, and rapid release when most polymer has degraded [22]. PCL is less used mainly because it bioerodes at slower rate than other aliphatic polyesters, but has the advantage of degrading in neutral species and not in acid byproducts [23].
Polymers for Coating
Coating of solid dosage forms allows facing up to a variety of formulation demands: taste masking, improvement of appearance, easy swallowing, prolonged stability and spatiotemporal control of drug release [24, 25].
Shellac is an excellent film-forming natural polyester resin secreted by insects. It consists in a complex mixture of aliphatic and alicyclic acids, which serves as moisture barrier of tablets and pellets due to its low permeability to water and oxygen [26, 27]. Shellac films protect drugs from degradation in the gastric environment. However, its use is limited by stability problems.
Cellulose ethers also exhibit good filmogenic features. Low-viscosity grades are suitable for aqueous film-coating, while higher-viscosity grades are used with organic solvents. HPMC films are gastrosoluble. Oppositely, ester derivatives of HPMC such as hypromellose acetate succinate or hypromellose phthalate can provide enteric films that resist prolonged contact with the strongly acidic medium, but dissolve in the mildly acidic or neutral intestinal environment. Cellulose esters, particularly acetate and acetate phthalate (CAP), are commonly applied to solid-dosage forms either by coating from organic or aqueous solvent systems, or by direct compression. CAP has the advantage of being compatible with most plasticizers [28].
Pectin, amylose, dextran and inulin provide coatings that degrade by enzymes of colonic flora. These polysaccharides are commonly combined with cellulose ethers or esters or synthetic polymers to obtain biphasic release profiles or colon-specific release [29–31]. Drugs conjugated to dextran (linear polymer backbone of α-D-glucopyranose units) show enhanced stability in the upper part of the gastrointestinal tract, but they can be absorbed in the colon [32].
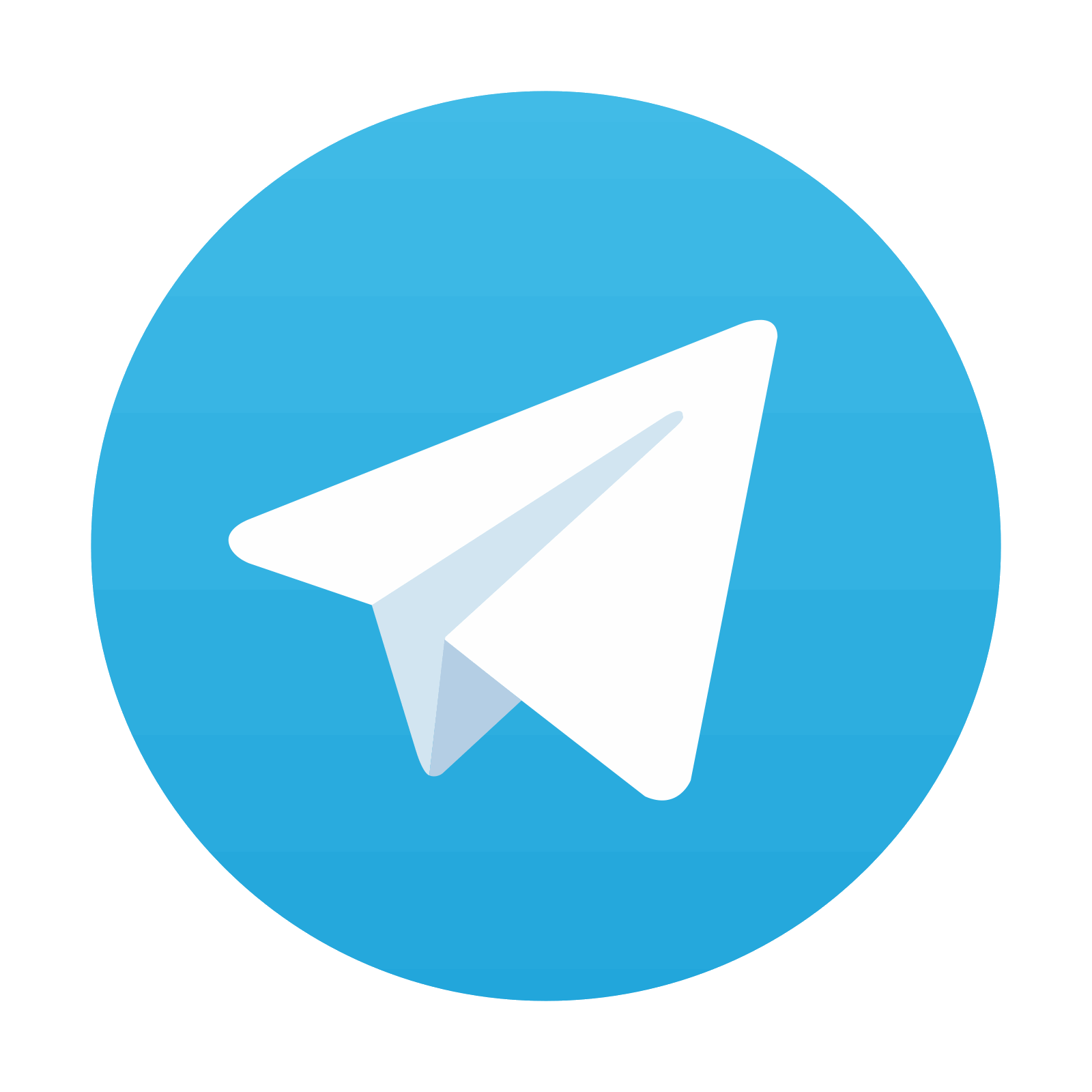
Stay updated, free articles. Join our Telegram channel
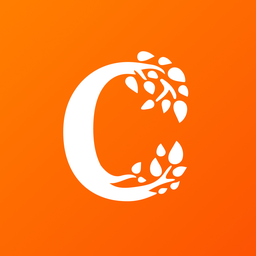
Full access? Get Clinical Tree
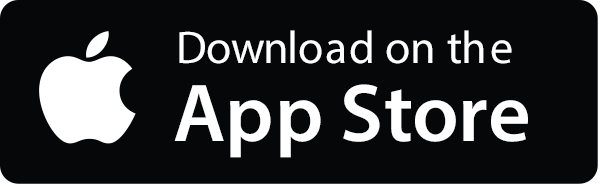
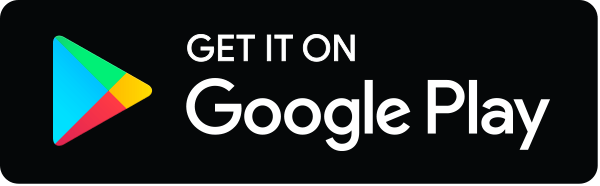