Scaffold
Manufacturer
Polymer
Coating
Drug
Resorption time (months)
Igaki-Tamai
Kyoto Medical
PLLA
None
None
24
BVS 1.0
Abbott Vascular
PLLA
PDLLA
Everolimus
24
BVS 1.1
Abbott Vascular
PLLA
PDLLA
Everolimus
24
DESolve
Elixir
PLLA
None
Myolimus
12–24
Amaranth
Amaranth
PLLA
None
None
3–6
ART18AZ
ART
PDLLA
None
None
3–6
REVA
REVA Medical
PTD-PC
None
None
24
ReZolve
REVA Medical
PTD-PC
None
Sirolimus
4–6
Ideal BioStent
Xenogenics
SA/AA polymer
Salicylate
Sirolimus
>12
However, comprehensive clinical requirements in terms of stent deliverability and stent mechanics compared to BMS can not all be fulfilled easily with polymeric scaffolds. As first Tamai et al. [98] reported their clinical experiences with an absorbable coronary polymer scaffold made from PLLA, the Igaki-Tamai scaffold. The Igaki-Tamai scaffold had a self-expanding zigzag helical coil design, where the self-expansion of this scaffold was assisted by balloon-expansion. The obtained results of this first study in humans demonstrated a low restenosis rate and a re-intervention rate of 10.5 % at 6 months [98]. The first clinically investigated fully absorbable DES is the bioresorbable vascular scaffold (BVS) by Abbott Vascular (USA). The BVS is based on a balloon-expandable PLLA scaffold and a PDLLA coating containing everolimus [134]. The first BVS generation (BVS 1.0) was tested in different clinical studies and was found to be safe [97]. The second generation (BVS 1.1) was enhanced regarding its radial strength, mechanical integrity and drug release kinetics with the purpose to establish a scaffold platform for the routine clinical practice and with the wish to be the fourth revolution in interventional cardiology [97]. Currently, scaffold developments of approximately 16 companies including many key and niche players such as the above mentioned and others are under consideration. Therefore, a lot of innovations can be awaited in this field in the future.
Polymers as Coating Matrices for Drug-Eluting Stents (DES)
As described in Chap. 2 DES consists typically of a stent platform, a drug and a polymeric drug carrier. For the optimization of the polymeric drug carrier and therefore for the effective prevention of delayed healing and hypersensitive foreign body reactions observed with first-generation DES advanced biocompatible permanent polymers, such as a biomimetic phosphorylcholine polymer (PC), the extremely biointert poly(vinylidene fluoride)-hexafluoropropylene (PVDF-HFP) or the BioLinx™ polymer customized regarding a optimized, sustained drug release profile, were used in second-generation DES coatings. Furthermore, biodegradable polymers, such as PLA and poly(lactide-co-glycolide) (PLGA), were studied to optimize DES coatings regarding their biocompatibility. Due to the fact that the polymeric coatings degrade over time and the DES transform into a BMS they are expected to cause lower stent thrombosis. Despite these refinements seen in the current polymer-based DES polymer-free DES have been investigated where the drug was embedded mostly into microporous or nanoporous metallic stent surfaces [15, 99]. This parallel development took place, because it is known that the degradation products of some biodegradable polymers are associated with tissue inflammation during the degradation process [44] and thus these coatings may also hold the risk to cause stent thrombosis [15]. Other disadvantages of polymer-based stent coatings could be that delaminations during the stent expansion (high plastic deformation) occur and their mechanical properties are impaired after sterilization due to the fact that the cleavage of chemical bonds leads to a post-crystallization and embrittlement of the polymer [99]. The main DES equipped with polymer coatings are summarized in Table 15.2. However, intense work on stent development has successfully led to DES the next generations of DES will further improve the endothelialization and enhance the arterial healing possibly using biocompatible, polymer-based abluminal or dual, side-selective coatings, as well as other innovative, polymer-free drug reservoirs.
DES | Manufacturer | Polymer coating | Coating thickness (µm) | Kind of polymer | Drug | Drug amount (µg/mm2) |
---|---|---|---|---|---|---|
Cypher | Cordis | PEVA, PBMA | 12.6 | Biostable | Sirolimus | 1.4 |
Taxus express | Boston Scientific | SIBS | 16 | Biostable | Paclitaxel | 1.0 |
Endeavor | Medtronic | PC | 4.1 | Biostable | Zotarolimus | 1.0 |
Endeavor resolute | Medtronic | BioLinx™ polymer | 4.1 | Biostable | Zotarolimus | 1.0 |
Xience-V | Abbott Vascular | PBMA, PVDF-HFP | 7.6 | Biostable | Everolimus | 1.0 |
Promus element | Boston Scientific | PBMA, PVDF-HFP | 6 | Biostable | Everolimus | 1.0 |
BioMatrix | Biosensors | PLA | 10 | Biodegradable | Biolimus A9 | 1.56 |
Polymers as Coating Matrices for Drug-Coated Balloons (DCB)
As alternative to DES DCB were developed since it was found that a single short exposure to PTX using non-ionic contrast media or balloon catheter coatings as carriers for local drug delivery provided similar protection from restenosis as the most efficacious DES [16, 101, 102]. Since these first preclinical observations more than 10 years ago different DCB concepts were proven (Table 15.3). Regarding their deliverability a clear inferiority of PTX-coated balloons using low-molecular excipients in comparison to uncoated balloon catheters was demonstrated [16]. Furthermore, it was stated that a PTX dose of 3 µg/mm2 is effective and tolerable for the DCB utilization [16, 103–105].
DCB | Manufacturer | Excipient | Drug | Drug amount (µg/mm2) |
---|---|---|---|---|
SeQuent Please | B.Braun | Contrast medium (iopromide) | PTX | 3.0 |
Dior II | Eurocor | Shellac | PTX | 3.0 |
Pantera Lux | Biotronik | BTHC | PTX | 3.0 |
Elutax | Aachen Resonance | 3-Layer design | PTX | 2.0 |
In.Pact Falcon | Medtronic Invatec | Urea | PTX | 3.0 |
While DES coatings are designed as a drug reservoir in order to allow a sustained drug release over a long time period, the development of DCB affords coatings which are robust enough to physically maintain the drug on the surface of the balloon during transit of the device through the vascular system and allow a rapid, uniform and efficient drug transfer to the vessel wall during a short balloon dilatation time. Coating additives of commercially available DCB, which all engage the drug PTX, are equipped in such a way to enhance the PTX transfer to the vessel wall [16, 106]. Some of them are well studied as e.g. the contrast agent iopromide [101], plasticizers such as n-butyryl tri-n-hexyl citrate (BTHC) [107] and urea [108] (Table 15.3). In addition, hydrophilic, polymer-based DCB coatings with high swelling and slippage properties could have a high potential and will be presented in more detail in section “DCB Equipped with Hydrogel-Based Coatings”.
Polymers for Transcatheter Aortic Valve Therapy
Due to the demographic development and increasing elderly population a growing group of patients will decline from surgical valve replacement, because they are too ill or weak to withstand the stress of invasive surgical treatments. In consideration of avoiding the need of open heart surgery and its associated risks, TAVI represents an ideal answer. Mostly, valve prostheses consist of a cobalt chromium alloy (balloon-expandable) or nitinol (self-expanding) stent structure where a tri-leaflet valve composed of porcine or bovine pericardium is mounted (Table 15.4).
Table 15.4
Exemplary overview of valve prostheses consisting of xenogenic pericardium
Prosthesis | Manufacturer | Stent | Valve |
---|---|---|---|
Sapien-XT | Edwards | Cobalt chromium alloy, balloon expandable | Bovine pericardium |
CoreValve | Medtronic | Nitinol, self-expanding | Porcine pericardium |
JenaValve | JenaValve | Nitinol, self-expanding | Porcine root valve (TA), porcine pericardium (TF) |
Portico | St. Jude | Nitinol, self-expanding | Bovine pericardium (TA), porcine pericardium (TF) |
Engager | Medtronic | Nitinol, self-expanding | Bovine pericardium |
Lotus | Boston Scientific, Sadra Medical | Braided nitinol | Bovine pericardium |
Although no perseverative anticoagulation is required biological valves based on xenogenic pericardium demonstrated a decreased durability due to the fact that they tend to calcify in comparison to mechanical valves [19]. Thus, functional synthetic valves were developed. Exemplarily noted is a novel nanocomposite material described by Rahmani et al. [20, 23] that composed of a polycarbonate urea urethane (PCU) as soft segment and a hard segment derived from polyhedral oligomeric silsesquioxane (POSS) nanoparticles through covalent bonding forming pendant chain functional groups. The POSS-PCU nanocomposite demonstrated superior mechanical properties compared to porcine and bovine pericardial tissues. Additionally, it possessed enhanced resistance to calcification and thrombosis with superior in vivo biostability [20]. Furthermore, textile, less fragile polyester-based leaflets were designed as alternative to xenogenic, biologic materials [21, 22]. Based on these examples can be stated that also synthetic, polymeric materials could be an alternative replacement for valve leaflets.
Surface Modification of Polymers Intended for Local Drug Delivery and Biofunctionalization
The Objective of Surface Modification for Polymers Used in Cardiology
Much effort is invested in the novel design and synthesis of polymers to be used as biomaterial for the fabrication of implants with appropriate mechanical properties, durability and functionality. In dependence of the application, requirements might differ considerably, ranging from high mechanical stress resistance especially interesting for endoprostheses to high transparency in ophthalmologic implants. Also in the confined field of cardiology, polymers with various properties are used in a wide range of applications. For instance, the pumping bladder in an artificial heart should withstand a millions of cycles without failure while a stent platform needs to allow for a high degree of deformation. These functions are generally governed by the bulk composition of the polymers. The biological response to the devices or the composing polymers is in contrast largely controlled by the surface chemistry and structure. The rationale for the surface modification of polymers is according to Ratner et al. [110] therefore straightforward: retaining the key physical properties of a biomaterial while modifying only the outermost surface to influence the biointeraction. Commonly observed interactions of any material with a biological system or system containing biomolecules cover adsorption or adhesion processes of proteins and bacteria or platelets as well as phagocytosis and fibrous encapsulation. Especially relevant polymer-blood, -cell and -tissue interactions in cardiology are in detail reviewed in Chap. 5. Effective surface modification of polymers should mediate these interactions with the purpose of improved tissue-interface related-biocompatibility without modifying the mechanical properties and functionality of the device. In principal there are two categories of surface modifications. The first involves the overcoating of an existing surface by coating, grafting or thin film deposition of a material having a different composition and properties than the bulk material. The second category involves the chemical altering of atoms, compounds or molecules in the existing surface. Both strategies are relevant for polymer surface modification in cardiology. While coating technologies are mainly applied for the provision of cardiovascular implants with a polymer-based drug-delivery function [section “Polymer-Based Local Drug Delivery (LDD) Systems”], chemical surface modification can be applied for both, drug delivery and stable functionalization with biomolecules (section “Biofunctionalization of Polymers”); all with the purpose of mediating the cell-implant interaction.
Polymer-Based Local Drug Delivery (LDD) Systems
Classification of Polymer-Based LDD Systems
In general, polymer-based drug delivery systems can be broadly classified into physically and chemically controlled systems. Diffusion-controlled drug release is an example of a physical mechanism that is based on the diffusion of a drug molecule through a certain coating or matrix, mostly of polymeric nature. Besides the benefit that the drug is fixed on the implant’s surface and relatively protected against mechanical stress, the thickness and type of polymeric coating allow a wide range of control over the drug release characteristics [111, 112]. In a further iteration of this approach, diffusion-controlled LDD systems can be divided into matrix and membrane systems, where the drug is either directly incorporated within or surrounded by the polymeric coating. Amongst others, these polymer-based LDD systems can be obtained by dip-coating and spray-coating techniques where the intended polymer and drug are either handled in the same solution, which might be a mixture of two solvents if needed, or separately for matrix and membrane systems, respectively. Polymers applied are rather hydrophobic to retard water interpenetration and thereby guarantee sustained drug release. If degradation of the device is desired, hydrolyzable polymers as polyesters with slower degradation than drug diffusion rate can be used for these systems. Table 15.5 provides an overview of polymers used in controlled drug release. Chemically controlled LDD systems offer another strategy for drug release. In these systems, drug release is achieved by degradation or swelling of the drug carrier or by cleavage of chemically bound drugs or biomolecules. For degradation-controlled systems polyanhydrides and poly(ortho esters), possessing highly labile groups that ensure rapid hydrolysis of polymer chains encountering water molecules and retarded water permeation by designing the polymers with hydrophobic monomer units, are used [113]. In contrast for LDD-systems, controlled via swelling, more hydrophilic polymers as e.g. hyaluronic acid are required. However, both systems, diffusion- and chemically controlled, provide drug release independently of their need, possibly leading to ineffectiveness of the LDD system at time of interest. Having this in mind, it would be highly beneficial if the drug is delivered by a system that senses the signal caused by disease, judges the magnitude of the signal and then acts to release the right amount of drug in response [114]. Here, stimulus-responsive hydrophilic polymeric networks as poly(acrylic acid) or poly(N-isopropyl acrylamide) hydrogels with swelling properties dictated by changes in pH [115, 116] or temperature [117–119], respectively, came into discussion. The induction of hydrogel swelling by such physical and chemical stimuli allows control over release of incorporated or underlying drugs. However, the cited stimuli are rather non-specific. An alternative are specific chemo-responsive hydrogels, which are designed to contain internal non-covalent interactions based on pendant ligands and complementary receptors [120]. These crosslinks can be broken by soluble ligand or receptor competitors, which are specific for certain diseases and can diffuse into the gel displacing the internal affinity crosslinks. Subsequently, the degree of crosslinking decreases and drugs can be released. Besides glucose-responsive hydrogels with concanavalin A—glucose affinity crosslinks [121], antigen-responsive hydrogels, where antibody–antigen pairs, chemically grafted to the hydrogel network serve as reversible crosslinkers, are discussed in literature. Several model systems have been established [120, 122, 123], while the best studied is probably the one of Miyata et al. [124–127], which is based on immunoglobulin G (IgG) antigens and corresponding antibodies grafted onto a poly(acrylamide)-(PAAm-)hydrogel, forming affinity crosslinks cleavable in the presence of free antigen. In an own study, we provided a thorough in vitro characterization of PAAm-hydrogels regarding drug permeability with the purpose of exploring the applicability of such hydrogels for implant-associated LDD systems [128]. Within this study, we were furthermore able to demonstrate an antigen-triggered release of bovine serum albumin as model drug from a model implant-associated LDD system. The continuation of work will now focus on the real implementation of such stimulus-responsive hydrogels to implant surfaces and the use of natural biocompatible polymers, particularly those in the poly(saccharide) family (e.g. hyaluronic acid and chitosan).
Table 15.5
Summary of commonly applied polymers in drug delivery (DD) systems
Polymer | Application |
---|---|
Chitosan | Biodegradable matrix for swelling-controlled DD |
Dextrane | Biodegradable matrix for stimulus-responsive DD |
Hyaluronic acid (HA) | Biodegradable matrix for swelling-controlled DD |
Poly(acrylic acid) (PAA) | Permanent matrix for pH-sensitive DD |
Poly(acrylamide) (PAAm) | Permanent matrix for stimulus-responsive DD |
Poly(anhydride) (PAH) | Biodegradable matrix for degradation-controlled DD |
Poly(n-butyl methacrylate) (PBMA) | Permanent matrix for diffusion-controlled DD |
Poly(ε-caprolactone) (PCL) | Biodegradable matrix for diffusion-controlled DD |
Poly(ethylene-co-vinyl acetate) (PEVA) | Permanent matrix for diffusion-controlled DD |
Poly(glycolide) (PGA) | Biodegradable matrix for diffusion-controlled DD |
Poly(hydroxybutyrate) (PHB) | Biodegradable matrix for diffusion-controlled DD |
Poly(lactide) (PLA) | Biodegradable matrix for diffusion-controlled DD |
Poly(N-isopropyl acrylamide) (PNIPAAm) | Permanent matrix for temperature-sensitive DD |
Poly(ortho esters) (POE) | Biodegradable matrix for degradation-controlled DD |
Poly(styrene-b-isobutylene-b-styrene) (SIBS) | Permanent matrix for diffusion-controlled DD |
Poly(vinylpyrrolidone) (PVP) | Permanent matrix for swelling-controlled DD |
Polymer-Based LDD in Cardiology
LDD entered the field of cardiology with the development of drug-eluting stents (DES) which revolutionized the treatment of coronary artery disease. Stents can generally be described as tubular scaffolds from a wire mesh or a slotted tube, which are either self-expanding or balloon-expandable. Typical biomaterials for stent manufacture are stainless steel and cobalt-chromium alloys for balloon-expandable stents, and nickel-titanium alloys (nitinol) for self-expanding stents [129]. DES are specialized vascular stents which allow the delivery of drugs in a controlled manner to arterial wall with the purpose to reduce or prevent in-stent restenosis as process of enhanced smooth muscle cell proliferation. As described the most DES consist of a polymeric matrix as drug carrier, which controls the drug release [130, 131]. Drug carriers used on the initial commercially available DES were permanent polymers as a blend of PEVA and PBMA in ratio of 50/50 % (w/w) (Cypher stent, Johnson & Johnson, USA) and the copolymer SIBS (Taxus stent, Boston Scientific, USA). These polymeric coating materials however raised the question about long-term biocompatibility. Therefore, the activities in DES development headed for more biocompatible and biodegradable materials as PLA [38, 132, 133] and copolymers, such as PLGA [134, 135], which are fully metabolized to carbon dioxide and water (Krebs cycle). These polyester-based drug reservoirs have the advantage that they degrade during or after the drug release so that they do not cause long-term foreign body reactions. In addition, degradable polymer blends of P(4HB) and PDLLA have been developed. Recently several design approaches to provide temporary absorbable stents, which may allow for restoration of vasomotion and long-term positive remodeling of the stented vessel [14], with an LDD-function are discussed. The first clinically available stent is equipped with a PDLLA-coating (BVS, Abbott, USA).
Biofunctionalization of Polymers
Techniques in Surface Activation
Due to the low abundance of available functional groups on the surface of most polymers, their surface activation is often a precursor to performing biofunctionalization in the sense of chemically attaching a bioactive compound. Techniques that modify surface properties by introducing random, non-specific groups or by coating the surface are less useful in bioconjugation to polymer surfaces. Used surface activation techniques should in contrast be tailored to introduce a specific functional group, which might be achieved amongst others by wet- and plasma-chemical processes, detailed in the following and in Table 15.6.
Table 15.6
Overview of polymers subjected to surface activation treatments
Surface treatment | Polymer | Generated function |
---|---|---|
Treatment with chromic acid | Poly(ethylene) (PE) | Oxygen-containing groups |
Poly(propylene) (PP) | ||
Acidic/basic hydrolysis | Poly(ε-caprolactone) (PCL) | Hydroxyl and carboxyl groups |
Poly(methyl methacrylate) (PMMA) | ||
Aminolysis | Poly(L-lactide) (PLLA) | Amine groups |
Poly(lactide-co-glycolide) (PLGA) | ||
Poly(methyl methacrylate) (PMMA) | ||
Poly(urethane) (PUR) | ||
Oxygen plasma | Polyamide (PA) | Oxygen-containing groups |
Poly(ε-caprolactone) (PCL) | ||
Poly(ethylene) (PE) | ||
Poly(ethylene terephthalate) (PET) | ||
Poly(L-lactide) (PLLA) | ||
Carbon dioxide plasma | Poly(ethylene) (PE) | Carboxyl groups |
Poly(propylene (PP) | ||
Poly(styrene) (PS) | ||
Ammonia plasma | Poly(ε-caprolactone) (PCL) | Amine groups |
Poly(L-lactide) (PLLA) | ||
Poly(styrene) (PS) | ||
Poly(tetrafluorethylene) (PTFE) | ||
Silanization | Polyamide (PA) | Amine-, epoxy-, vinyl-groups |
Poly(ε-caprolactone) (PCL) | ||
Poly(ethylene terephthalate) (PET) | ||
Poly(L-lactide) (PLLA) | ||
Poly(methyl methacrylate) (PMMA) | ||
Poly(tetrafluoroethylene) (PTFE) |
Wet–chemical surface activation. In wet-chemical surface modification, a material is treated with liquid reagents to generate reactive functional groups on the surface. In contrast to plasma and other energy source surface modification techniques, this method has a general higher penetration depth and therefore modification of three-dimensional scaffolds within pores is often feasible in a simple way [136]. For instance, oxygen-containing moieties were introduced to PE [137, 138] and PP by treatment with chromic acid and potassium permanganate in sulfuric acid [139, 140]. Carboxyl and hydroxyl groups were generated by base and acid hydrolysis of PMMA [141], and PCL [142] with concentrated sodium hydroxide and sulfuric acid. One has to note that hydrolysis time of polyesters needs to be thoroughly optimized in order to retain the properties of the bulk polymers. Subsequent conversion of the functional groups to isocyanate groups by treatment with 4,4′-methylenebis(phenyl isocyanate) allowed formation of terminal amino groups by addition of ammonia. In an own study, we have already applied this activation procedure for the immobilization of acetylsalicylic acid and vascular endothelial growth factor (VEGF) to PCL [142]. Generation of primary amines is also possible by wet-chemical treatment without the intermediate step of hydrolysis. For instance aminolysis with various diamines including hydrazine hydrate, 1,6-hexanediamine, ethylenediamine, and N-aminoethyl-1,3-propanediamine, as well as lithiated diamines [7, 19, 22–28] has been applied to introduce amines to PMMA [143, 144], PUR [145], PLLA [146, 147], and PLGA [148]. The simple these methods are, they are however non-specific and the degree of surface functionalization might therefore not be repeatable between polymers of different molecular weight, crystallinity, or tacticity. Moreover, hazardous chemical waste might be generated [149]. A clean alternative are ionized gas treatments, first of all plasma-chemical modifications, described in the following.
Plasma–chemical surface activation. In contrast to the above described wet-chemical methods plasma treatments can provide modification of the top nanometer of a polymer surface without using solvents or generating chemical waste and with less degradation and roughening of the material than many wet-chemical treatments [136]. The type of inserted functional groups can be varied by selection of the plasma gas (Ar, N2, O2, H2O, CO2, NH3) and operating parameters (pressure, power, time, gas flow rate) [136]. For instance, surfaces of PCL [142, 150], PLLA [147], PA [18], PE [137], and PET [151] were provided with oxygen containing functional groups by oxygen plasma exposition. Moreover, carbon dioxide plasma could be used to introduce carboxyl groups to PP [152], PS [153], and PE [152]. The generation of amine groups was in addition performed by ammonia plasma to PTFE [154] and PS [153]. In own studies we treated PCL [142] and PLLA [147] by ammonia plasma and qualified the generation of amine groups via fluorescent labelling. Results reveal less functional groups on these surfaces in comparison to chemically activated surfaces, which is in good agreement with the well-known low plasma penetration depth of only 10 nm. However, no correlation with the efficiency of subsequent immobilization of biomolecules could be observed [155]. Besides a standalone technique, plasma treatments are often used as a precursor to other surface modification techniques, as for example plasma activation followed by silanization, as in detail described in the following.
Surface activation via silanization. Although surface modification using silane monolayers is primarily applied on glass and silicone substrates as a means to couple an organic polymer, this is certain to be an emerging field in research for polymer surface modification [136]. Because the target surface functional group in glass or silicon silanization is a hydroxyl, silanization can be applied to polymer surfaces, which have been hydroxylated by for instance treatment with oxygen plasma. As organosilanes with various end functionalities including amine, epoxy and vinyl groups are available, silanization offers the potential of modifying polymer surfaces with a bunch of functional groups for different application. Moreover, self assembled monolayers, produced by silanization, provide a nearly crystalline and hence more defined surface functionalization than typical wet-chemical or ionized gas functionalization techniques [156]. In own studies we used organsilanes with epoxy and amine head groups for the chemical attachment of hyaluronic acid to PA [18] and VEGF [142] and antibodies [147] to polyester surfaces, respectively.
Techniques in Biomolecule Immobilization
Among the numerous reported methods for immobilizing biomolecules to surfaces physical adsorption via van der Waals or electrostatic interactions, physical entrapment within hydrogels, ligand–receptor pairing (as in biotin–avidin) and covalent attachment via cleavable or non-cleavable bonds are most commonly used (Fig. 15.1). In dependence of the applied immobilization protocol a short-term and long-term localization of the biomolecule on the implant surface can be achieved [110].
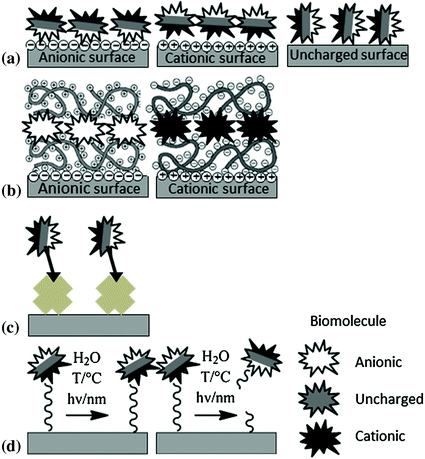
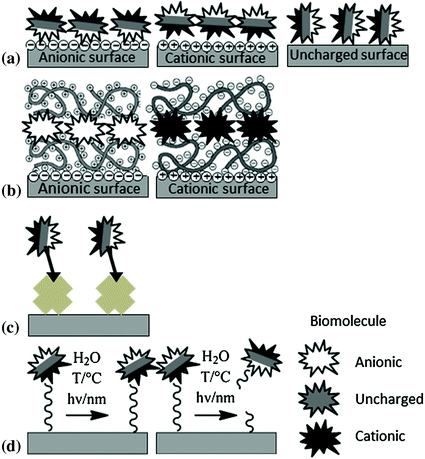
Fig. 15.1
Biomolecule immobilization strategies: a physical adsorption, b physical entrapment within hydrogel, c ligand–receptor pairing, and d covalent attachment
Thus, biofunctionalization can be either used to provide the implant with a drug delivery or a stable novel surface functionality. In an own study, we could show this wide range of modification possibilities by VEGF immobilization and delivery from PLLA surfaces [157]. Observed release profiles differed in dependence of the applied immobilization method. Fastest release was observed for VEGF entrapped in a PAA hydrogel, followed by physically adsorbed VEGF and covalently attached VEGF via hydrolyzable bonds with the slowest release rate. The release of physically adsorbed or entrapped VEGF is diffusion-controlled and starts immediately after insertion into the elution medium. Chemical attachment however affords the cleavage of covalent bonds prior to liberation of biomolecules. This was achieved in that case by the formation of ester bonds between the side chain hydroxyl groups of VEGF and N,N-disuccinimidyl carbonate-activated surface amino groups on the PLLA surface.
As detailed above most of biofunctionalization methods afford a previous surface activation, which might be combined with the grafting of an intermediary spacer between the surface and the biomolecule for multiplication of available functional groups on the surface. Here, multifunctional spacers as poly(ethylenimine) or dendrimers with a wide range of terminal functional groups in defined quantity offer a way to increase this surface functionality [157, 158]. In addition, maintenance of biological activity is very important for hydrophobic polymer surfaces as these are often associated with non specific adsorption and denaturation. Besides the integration of hydrophilic PEG spacer [159], which effectively shield the compound from these issues, we investigated in an own study the inclusion of the peptide spacer (GGAP)4 on PLLA surfaces. By this we reached efficient prevention of non-specific protein adsorption and enhanced bioactivity of subsequently covalently attached anti-CD34 antibodies [147].
A prerequisite for successful biomolecule immobilization is of course moreover that the specific functionality imparted to the inert surface must be compatible with the reactive sites on the compound to be covalently attached to that surface. While for adsorption processes the introduction of charged groups or simple modification of hydrophilic properties should be enough, covalent immobilization affords defined functional groups, including thiol, aldehyde, carboxylic acid, hydroxyl, and primary amine groups. These can be directly used for biomolecule attachment. However, most cases apply additional crosslinking agents, which can link the bioactive compound directly to the functionalized substrate (zero-length crosslinkers), or introduce themselves a spacer of several angstroms. Moreover, they can be stable or introduce hydrolyzable bonds in order to allow for biomolecule delivery from the surface. A detailed description of these agents and the associated chemistries can be found in Bioconjugate Techniques, by Hermanson [160].
Biofunctionalization of Polymers for Application in Coronary Vascular Intervention
In the field of cardiology, intensive research on biofunctionalization of polymers has been done since decades to develop novel surface designs for all types of endovascular implants in order to modulate their behavior in contact with blood and improve their bio-and hemocompatibility. Even prior to the development of DES this issue has been addressed by the establishment of stents, being coated with the anticoagulant heparin (e.g., Palmaz-Schatz stent [161, 162], Wiktor stent [163], and Jostent [164]), fibrin [165], cellulose [166], and phosphorylcholine (BiodivYsio stent [167]). Nowadays more attention is however drawn to the biofunctionalization of the polymer coating enabling activation of a cascade of biological processes that eventually regenerate or replace a functioning endothelium, referred to as prohealing approach. Materials promoting in situ endothelialization of vascular implants by capturing in vivo circulating endothelial progenitor cells (EPC) with the capacity to differentiate into mature endothelial cells (EC) and repopulate areas of vascular injury and endothelial disruption [168, 169]. The selection of the optimal target on the EPC surface in combination with an ideal capture molecule immobilized on the implant surface, which is decisive for the success of in situ endothelialization, is however still a challenge. A stainless steel-coated stent with a polysaccharide matrix covered by murine monoclonal anti-human antibodies directed towards the EPC surface marker CD34 is probably the most studied “EPC capture stent” nowadays. This Genous R-stent, developed by the company Orbus Medical Technologies in collaboration with Kutryk’s group, has achieved CE approval and is commercially available since 2010. Although CD34 is not a specific marker of EPC, as only a small portion of the CD34-positive cells are actually EPC [170], first human clinical investigations of this technology demonstrated its safety and feasibility for the treatment of de novo coronary artery disease [171] and the long-term promotion of significant late regression of neointimal hyperplasia [172, 173]. Unfortunately, in spite of these initial promising clinical outcomes, following randomized clinical trials comparing the Genous R-stent with BMS (GENIUS STEMI) [174] and DES (TRIAS) [175–177] evidenced disappointing results with regard to late luminal loss. These results let to a general discussion of the Genous’ clinical efficacy. As reaction to this discussion, novel surface modification strategies in order to increase availability of immobilized compounds and thereby biofunctionality of the modified surface and more selective capture molecules are searched. In this context, further antibodies than CD34 (e.g., anti-KDR) [178], against EPC selected aptamers [179], and materials mimicking the extra cellular matrix (ECM), are in focus of current research. From the plethora of insoluble and soluble macromolecules contained within ECM, the fibrillar protein collagen is probably the most commonly used coating on for example commercially available polyester vascular grafts [180]. Furthermore, besides the use of integer ECM components, various ECM peptide sequences as functional domains of proteins, glycoproteins, and proteoglycans have been isolated and grafted on biomaterials to control cell behavior, for example, REDV [181], PHSRN [182], RGD, and GRGDSP from fibronectin [183], laminin-derived recognition sequences, IKLLI, IKVAV [184] LRE, PDSGR, RGD, and YIGSR, and collagen type I derived sequence, DGEA [185]. However, these peptides, nicely reviewed by De Mel et al. [186], are not cell-selective as they bind to integrins, present on many cell types. To overcome this risk of low cell selectivity, Veleva et al. [187] used a combinatorial peptide library and phage display technique to isolate peptides, being more selective for human blood outgrowth endothelial cells (HBOEC). Indeed, selectivity of terpolymers, covalently coupled with these peptides, could be demonstrated for HBOEC in serum-free medium. The application of a coating combing both, an integer ECM component with functional peptide domains, might hence be very promising with regard to the creation of an optimal cell environment and thereby suppression of the foreign body reaction.
Biocompatibility of Polymers Used for Cardiovascular Applications
Interactions of Tissue- and Blood-Cells with the Polymer Surface Material
Diagnostic and therapeutical treatments implicate the contact between tissue, blood and the implanted material. In the cardiovascular field, a variety of biomaterial is implanted in heart and vessels, such as catheters, stents, heart valves and sondes for pacemakers and defibrillators. Using polymers for new technologies has been a revolutionary advance in the therapy of cardiovascular disease [47]. Nevertheless, there is increasing evidence that the polymer coating could be responsible for adverse effects (e.g. in-stent-restenosis, stent thrombosis, chronicle foreign body reactions). Therefore, a feasible biocompatible material should provide a complete re-endothelialisation of the surface, less thrombogenicity as well as anti-inflammatory properties in order to improve clinical outcomes.
Implantation of a polymer device into a vessel or tissue causes a complex inflammatory response inducing adhesive interactions between vascular cells (blood and tissue cells). During stenting, the endothelial layer is partially or completely destroyed. In animal experiments of vascular injury, denudation of endothelial cells results in platelet deposition followed by neointima formation [188–190]. In addition, complete coverage of endothelial cells is associated with attenuation or even stop of the growth of neointima from smooth muscle cells in the injured arterial segment [191, 192]. A poor endothelialisation promotes platelet aggregation, thrombus formation and stent-thrombosis. Therefore, the process of re-endothelialisation on the surface material requires sufficient biocompatible qualities of the underneath laying polymer surface. Recent studies have been demonstrated that proliferation, viability and function of endothelial cells, e.g. production of endothelial NO synthase, are dependent on the polymer surface [193, 194].
After stenting smooth muscle cells undergo complex phenotypic changes including migration and proliferation from the media towards the intima, and transition from a contractile to a synthetic phenotype [195]. Additionally, the release of cytokines and growth factors from white blood cells can induce increased smooth muscle cell growth and accumulation within the intima (neointima formation) leading to in-stent-restenosis.
Platelet adhesion and leukocyte rolling on injured endothelium are key events of this multistep process leading to platelet-leukocyte interaction, aggregation and activation of the coagulation cascade. Leukocyte rolling is mainly mediated by selectins (e.g. P-selectin) which interact with the leukocyte PSGL-1 receptor and by integrins (e.g. Mac-1) [196]. In terms of hemocompatibility, it is conceivable that slow rolling supports adhesion strengthening and spreading of polymorphnuclear cells and thus worsening hemocompatibility. Following monocyte adhesion and transmigration mediated by integrins, monocytes and monocyte-derived macrophages then secrete proinflammatory cytokines. These reactions can lead to chronic inflammatory responses. Chronic inflammation has been described as foreign body reaction where monocytes, macrophages and foreign body giant cells are present at the biomaterial interface for longer than 2 weeks [197]. Recent data from Busch et al. suggest differences in leukocyte activation and monocyte adhesion among various polymers (unpublished data). An explanation for this observation could be that biomaterials can modulate monocyte paracrine interactions to influence monocyte adhesion and viability [198]. Cohen et al. [199] demonstrated differences in monocyte activity on poly(ethylene glycol) hydrogels, poly(dimethyl siloxane), and tissue culture polystyrene in cultures with conditioned medium priming. Khandwekar et al. compared leukocyte adhesion on bare PCL surfaces and modulated PCL surfaces. They showed that leukocyte adhesion on bare PCL could even be more reduced when converting to a heparin-modified PCL [200].
Although red blood cells (erythrocytes) play only a minimal role in wound healing and blood-biomaterial interactions, the contact of red blood cells with the material can lead to hemolysis. Hemolysis is the breakage of the erythrocyte’s membrane with the release of intracellular hemoglobin. Normally, red blood cells live for 110–120 days. After that, they naturally break down and are removed from the circulation by the spleen. Some diseases and medical devices cause red blood cells to break too soon requiring the bone marrow to accelerate the regeneration of red blood cells (erythropoesis). Medical devices for hemodialysis, heart-lung-bypass machines or mechanical heart valves induce more hemolysis than smaller implants like stents or catheters [201].
Testing Bio- and Hemo-compatibility of Polymers
Biocompatibility determines the interface reactions of blood and tissue cells with the surface of the biomaterial. In this context, the term “hemocompatibility” mainly refers to blood component reactions during contact with the biomaterial [202]. It is of great importance to test various polymer characteristics in detail before developing the elaborated design for in vivo application. Over the past years, a variety of methods have been established to determine hemo- and biocompatibility of polymer implants. In this chapter a summary of standardized and new methods is provided.
Complementary Perfusion Systems
Cell interactions with polymers are usually studied using cell culture techniques. In vitro evaluation of surface materials by directly seeding endothelial cells (ECs) and smooth muscle cells (SMCs) onto the biopolymers represents a common procedure to assess biocompatibility and cytotoxicity as well as cell morphology [203–205]. In this context, most in vitro studies analyzing the impact of material on tissue cells involve experiments under static conditions. However, in healthy vessels especially ECs are exposed to the blood stream. This pulsatile, laminar blood flow exerts shear stress on vascular endothelium, which induces anti-apoptotic signals and maintains an anti-inflammatory and non-thrombotic endothelial phenotype compared to ECs cultured under static conditions [206, 207]. Cell culture experiments represent a model in which one can study specific mechanisms involved in the biologic responses of blood and tissue cells to materials. During the last decades a variety of techniques has been developed to investigate vascular biology under hemodynamic conditions.
Parallel Plate Flow Chamber. The parallel plate flow chamber (PPFC) is a design most frequently used to study vascular cells under flow conditions. Here, cell culture media is circulated through the chamber at adjustable flow rates creating a defined laminar flow (Fig. 15.2).
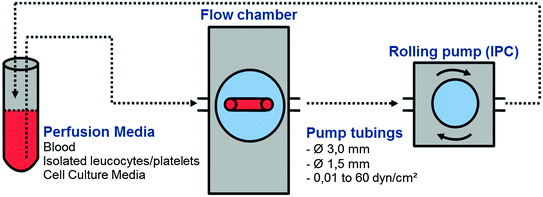
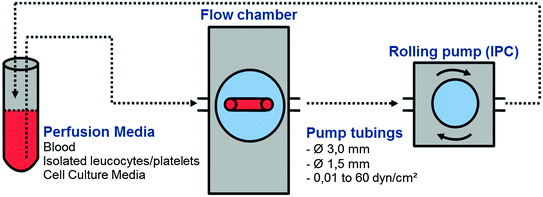
Fig. 15.2
Schematic diagram of an in vitro perfusion system for laminar shear stress conditions. Flow chamber contains the biomaterial with cultivated cells (e.g. HUVECs). Perfusion with full blood, isolated leukocytes, platelets or cell culture media. Rolling pump applies various volume rates. After perfusion, the perfused cells on the biomaterial (e.g. proliferation, vitality, PCR, Western blotting) and the supernatant (e.g. flow cytometry, ELISA) should be analyzed
A typical flow chamber consists of a polycarbonate corpus (bottom), a silicone gasket, which defines height and length of the flow channel (side walls) and a glass cover slip (top), which can be replaced by polymer foils. Under static cell culture conditions, the coverslip can be seeded with the cells of interest prior to the flow experiment. Additionally, these flow chambers are designed to allow fluorescence microscopic observation of a perfused cell layer. In this context PPFCs are suitable to measure the kinetics of cell attachment, detachment, and rolling on surfaces under flow conditions. Therefore, a range of studies refers to the adhesion of leukocytes and platelets on endothelial cells [208, 209]. Recently, Busch et al. [193] published a study which assessed a range of endothelial parameters in interaction with different stent materials using an in vitro perfusion system. However, these kind of experiments are limited by the rectangular geometry of the flow channel and do only allow for the investigation of cell monolayers over several hours.
Live Cell Imaging. To investigate the influence of the substrate regarding endothelial cell morphology in particular, it might be advisable to culture endothelial cells under flow conditions for several days. For that purpose, a specially designed sticky–slide (ibidi) can be utilized [210]. Different substrates can be mounted to the bottomless channel slide, which has been developed for microscopic applications. The slide can then be connected to the ibidi pump system and kept sterile for several days in a conventional incubator. Additionally, for example the cytoskeleton can be visualized and changes in morphology due to the substrate can be monitored by microscopic methods.
Tube Chamber System. To overcome the limitations of 2D cell culture models vessel-simulating bioreactors have been developed. Typically, a 3D bioreactor consists of a graft with an individual culture media reservoir to allow a transversal gas and media exchange between the perfused graft lumen and the outer chamber volume which simulates the interstitial tissue liquid. The porous graft (e.g. silicone, PTFE) is seeded with cells and the culture media is perfused through the construct. This procedure allows the in vitro cultivation of endothelial cells under fluid flow and results in a synthetic vessel [210, 211]. A special modification of a vessel-simulating flow-through cell has been developed by Neubert et al. and is particularly suitable for the evaluation of drug release and distribution from drug-eluting stents [212–214].
Cell Adhesion, Proliferation, and Cytotoxicity
To investigate cell material interactions, cultured cells are seeded on the polymer surfaces of interest and the extent of cell adhesion, proliferation, and viability is measured by standardized assays. Although in vitro experiments do not entirely reproduce the whole range of cellular responses to the implanted material, these methods provide a level of quantification which cannot be easily obtained in vivo.
Cell adhesion. For most applications the adhesion of cells is of fundamental interest being the initial event of cell attachment. A simple method to quantify adherent cells is to incubate cells over a surface for a period of time subsequently followed by the detachment of loosely adherent cells by gently washing the surface. Remaining cells can be labeled by fluorescent dyes and quantified using fluorescent measurements. Cell adhesion assays are also frequently used to assess monocyte or platelet adhesion on polymeric surfaces [215, 216]. In this context Hezi-Yamit et al. [217] did show that polymer hydrophilicity should be considered as a parameter to assess the biocompatibility of polymer surfaces. They showed that hydrophobic polymers such as PBMA or SIBS promote the adhesion of inflammatory activated monocytes while more hydrophilic polymers (e.g. PC (Phosphorylcholine) polymer) lead to less pro-inflammatory responses.
Cell proliferation. Endothelial growth is an essential factor after implantation. A continuous and healthy endothelial layer only prevents inflammatory and thrombotic events on the vessel wall. Besides, a healthy endothelium is crucial for regulating smooth muscle cell growth [218, 219]. Cell proliferation can be assessed by commercially available BrdU ELISA assay kits. On the basis of the study by Busch et al. [193] proliferation of ECs on hydrophobic permanent polymers (PEVA, PBMA) as well as biodegradable polymers [PLLA, P(4HB)] is reduced, whereas the polymeric blend PLLA/P(4HB) promotes EC growth. Data from this study do also show that SMC proliferation is influenced in the same way as EC growth and none of the polymers tested is able to enhance endothelial proliferation by simultaneously reducing smooth muscle cell growth.
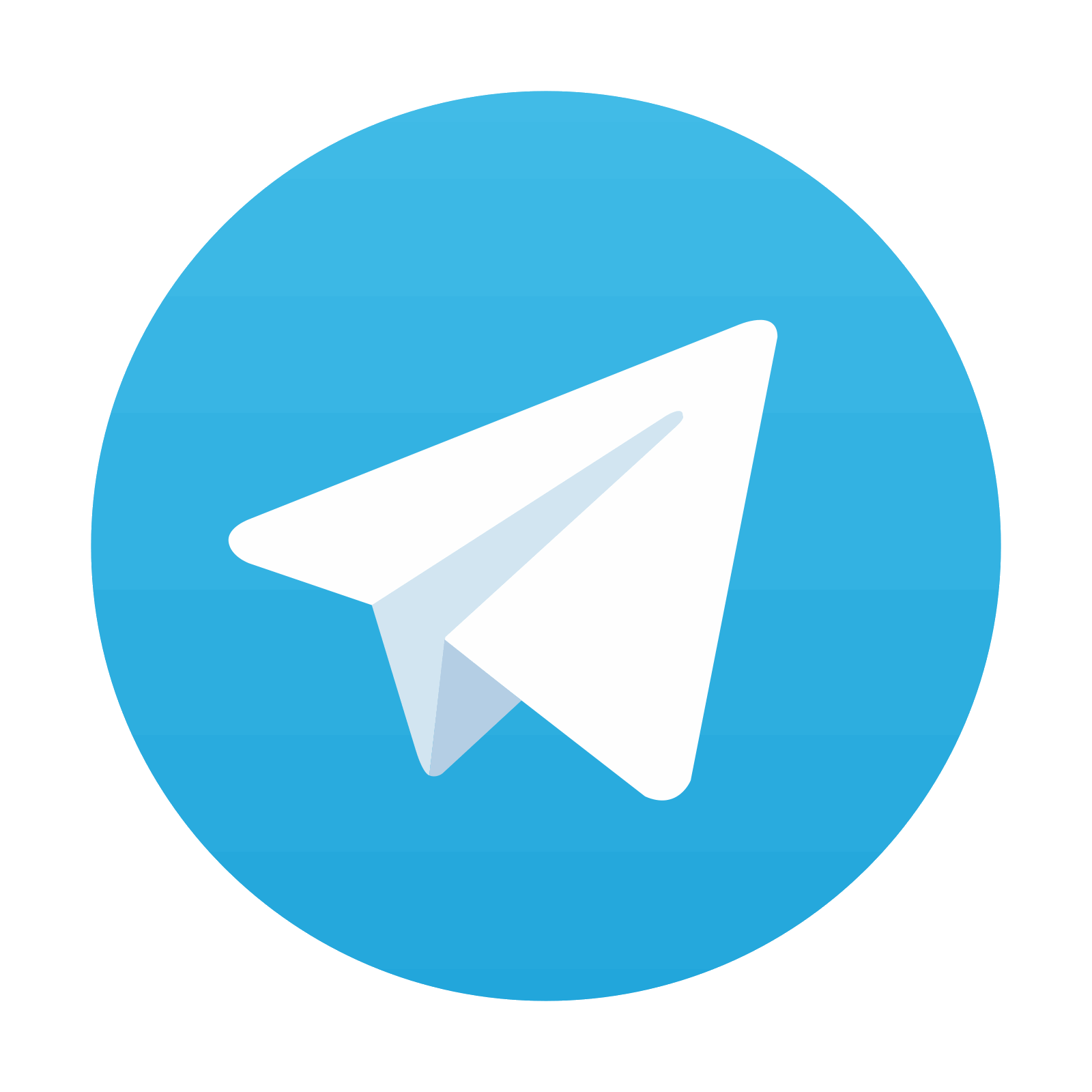
Stay updated, free articles. Join our Telegram channel
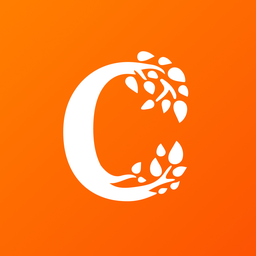
Full access? Get Clinical Tree
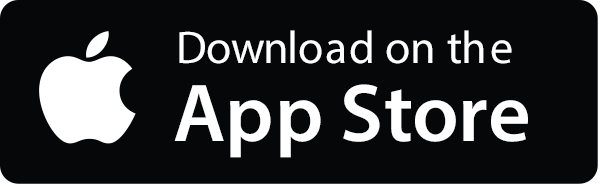
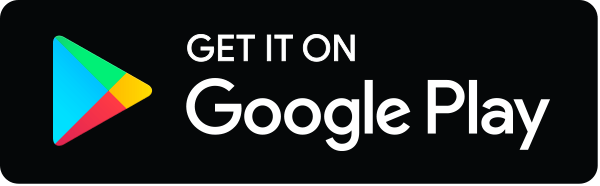