Plasma Sterilization
Akikazu Sakudo
Hideharu Shintani
Yagyu Yoshihito
The term plasma, referring to ionized gas, was first coined by Irving Langmuir in 1927 and was so named because it reminded him of blood plasma.1,2 Since the 1970s, different types of plasmas have been recognized to inactivate microorganisms. In the early 1990s, commercial instruments that use plasma during sterilization of medical devices became available. For more than a decade, plasma medicine, which refers to the application of plasma for biological decontamination as well as for therapeutic purposes, has undergone rapid development. These applications include the “plasma” treatment of blood plasma, such as argon plasma coagulation (APC).3 This terminology for “plasma” might cause confusion, which is why “gas plasma” is often used instead. In this chapter, because we do not deal with the treatment of blood, the term plasma is used throughout to refer to an ionized gas.
Diverse applications of plasma disinfection have recently been reported in the fields of medicine,4 dentistry,5,6 agricultural,7,8 and environmental science9,10,11 (Figure 34.1). Indeed, numerous scientific papers and patents over the past 20 years cite the microbicidal effect of plasma. Commercialized patents of plasma sterilization describe the use of oxidizing agent gases such as hydrogen peroxide12 and peracetic acid13 as well as ozone (O3) and chlorine dioxide (ClO2).14 However, it should be noted that these instruments are not true plasma sterilizers because the sterilization factor is the oxidizing agent gases rather than the plasma. In the case of the widely used hydrogen peroxide gas plasma sterilizers STERRAD (Advanced Sterilization Products, Irvine, California), common cycle conditions clearly show that the plasma is only generated following exposure and removal of the gas by a vacuum.15 In addition there was little or no contributory microbicidal activity in the presence and absence of plasma.16 The D-value (decimal reduction time: the time required for killing 90% [1 log] of the exposed microorganisms) for 1 mg/L of hydrogen peroxide gas against Geobacillus stearothermophilus was about 1 minute, whereas the estimated D-value in the presence of plasma at 300 W at the same concentration was 5 minutes.17 Therefore, the observed antimicrobial effect of STERRAD is due to the hydrogen peroxide gas and not the plasma. However, in such processes, the plasma is important because it may be involved as a heating mechanism and can aid in the removal of hydrogen peroxide (gas and liquid) residues from the sterilizer load, thereby making the contents safe. Furthermore, US Food and Drug Administration (FDA) issued a toxicity safety alert for hospital devices using a different plasma-based system, which involved peracetic acid gas exposure followed by plasma generation.18,19 Although this system has proven microbicidal activity, there were potential problems associated with compatibility of the device as well as toxicity issues.20
Interestingly, the authors’ studies have revealed that performance comparison of endotoxin inactivation showed that plasma of nitrogen (N2) gas (Figure 34.2) was superior to hydrogen peroxide gas plasma using STERRAD (Table 34.1).22 These findings prompted the authors to further study the potential use of this technology to ascertain whether it could elicit a broad spectrum of microbicidal activity against bacteria (including bacterial spores), viruses (enveloped and nonenveloped viruses), and fungi as well as the inactivation of prions and toxins (endotoxins, bacterial toxins, and fungal toxins). Our findings clearly showed that plasma sources could be suitable for these applications. Thus, plasma-based technologies can be applied not only for disinfection/sterilization but also for cleaning.23 In addition some recalcitrant forms of bacteria in the environment, including biofilms, could be inactivated by the plasma. As well as N2, plasma may be generated from a range of different gases including oxygen (O2); carbon dioxide (CO2); mock air (mixture of 20% O2 and 80% N2); and noble gases such as argon (Ar), helium (He), neon (Ne), krypton (Kr), and xenon (Xe) and mixtures thereof. The plasma generated from these gases caused inactivation of microorganisms, although the efficiency is dependent on the type of gas used.24
![]() FIGURE 34.1 Recent applications of plasma technology for disinfection and decontamination. For a color version of this art, please consult the eBook. |
![]() FIGURE 34.2 A representative nitrogen gas plasma instrument, BLP-TES (Bi-polar and Low-pressure Plasma-Triple Effects Sterilization) device. A, Photograph of the nitrogen gas plasma instrument (BLP-TES device; NGK Insulators, Ltd, Komaki, Japan). The BLP-TES device produces nitrogen gas plasma using a fast high-voltage pulse applied by a static induction (SI) thyristor power supply at 1.5 kpps and 0.5 atm. B, Photograph of the blue luminescence observed upon operation of the BLP-TES device. For a color version of this art, please consult the eBook. Reprinted from Sakudo et al.21 Copyright © 2016 Elsevier. With permission. |
|
Overall, plasma disinfection/sterilization can be used in three ways: (1) plasma is directly generated at the site of application (direct treatment of plasma), (2) plasma is generated at a remote site and transferred to the target site (indirect treatment of plasma by afterglow), and (3) plasma-treated solutions are used for disinfection/sterilization (solutions used as disinfectants are often referred to as “plasma-activated water [PAW]”25 or “plasma-treated water [PTW]).”26,27 Plasma-treated solutions known as “plasma-activated medium (PAM)”,28 “plasma-stimulated medium (PSM),”29 “plasma-treated phosphate-buffered saline (pPBS),”30 or “nonthermal plasma-conditioned media (NTP media)”31 have been reported to inactivate cancer cells but not healthy cells under certain test conditions. These solutions may also be used for therapeutic tests in the laboratory. In addition several cold plasma sources were certified as medical devices and have been used for the treatment of chronic wounds as well as other skin diseases.32,33,34 Several groups have developed devices for such clinical purposes including kINPen,32,33,34,35 Plasma-Derm,36 and MicroPlaSter.37 Because this book focuses on disinfection/sterilization, we would not deal with these plasma therapies in any further detail. If readers are interested in this issue, they should refer to some recent excellent reviews.38,39,40
In this chapter, we summarize the fundamentals of plasma and focus on plasma disinfection/sterilization of various microorganisms using nonoxidizing agent (or essentially inert) gases such as air, N2, O2, or noble gases.

Plasma is known as the fourth state of matter and comprises a gas containing electrons and charged particles.41 When energy is applied, matter changes state from solid to liquid and then from liquid to gas. When additional thermal energy is applied to the gaseous matter, the constituent molecules (or atoms in the case of a noble gas) collide with greater frequency and kinetic energy, resulting in a loss of electrons and the formation of an ionized state. This ionized gas is called plasma. The number of charged particles and the number of electrons are nearly equal in a plasma because positive ions and electrons are always generated in a pair during ionization, and a plasma is in an electrically quasi-neutral state, at least from a macroscopic perspective. Plasma has a distinct property in which physical, chemical, and electrical actions are mixed and interact in a composite manner. First, plasma is a gas that contains charged particles and therefore exhibits conductivity. Second, there is chemical activity, which makes the plasma highly reactive. Third, plasma glows and therefore can be used as a light source. Final, plasma has a high temperature, and the particles possess significant kinetic energy. Of particular relevance, plasma sterilization takes advantage of the plasma property in which plasma is able to generate highly reactive chemical species at a low temperature. Generally, using electrical energy is simpler and more efficient than using thermal energy. Acceleration in an electric field is made possible because the electrons are negatively charged. Plasma can be generated from ionizing neutral particles by providing kinetic energy that is greater than the ionization potential.
Aside from electrons and positive ions, other species including atoms, molecules, and neutral particles without charge, such as radicals, exist within the gas in a plasma state.42 A characteristic indicator of plasma is the degree of ionization β. Assuming an electron density ne, ion density ni, and neutral particle density nn, these indicators are defined as shown in equation (1).

A plasma with 100% degree of ionization is called a completely ionized plasma; ionization of several percentage or higher is called strongly ionized plasma; and a degree of ionization (0.1% or below) where neutral particles occupy the majority of space is called weakly ionized plasma. The properties of plasma are greatly dependent on the pressure. Because the area near the atmospheric pressure is highly dense, intense collisions between electrons, ions, and neutral particles take place and kinetic energy of the particles are exchanged. The kinetic energy of a gas is determined by absolute temperature only. If the
temperatures of electrons, ions, and neutral particles are Te, Ti, and Tn, respectively, then Te
Ti
Tn in an atmospheric plasma. A state in which each temperature is equal is called thermal equilibrium plasma, or simply, thermal plasma. However, plasma generated under a low pressure (less than a few hundred Pascal) has a low density of particles, and Te >> Ti
Tn because electrons do not lose kinetic energy through collisions. Plasma in a thermally nonequilibrium state is referred to as nonthermal equilibrium plasma or low-temperature plasma.
temperatures of electrons, ions, and neutral particles are Te, Ti, and Tn, respectively, then Te



Recent research in the field of medicine and bioscience involving the application of plasma technology has resulted in the development of pulse power sources that do not heat up under atmospheric pressure as well as atmospheric pressure plasma jets that use alternating current high-voltage discharge of several kilohertz and several kilovolts in an He gas.43

In the air, there are ions of particles and electrons generated under the influence of radiation from cosmic rays and from the natural world. These electrons are known as accidental electrons (initial electrons) and play an important role in initiating the generation of plasma.44 If voltage is applied between electrodes to generate plasma, accidental electrons (initial electrons) that accidentally existed between the electrodes are accelerated by the electric field, generating new ions and electrons through collisions with other particles. Similarly, newly liberated electrons generated by ionization are also accelerated by the effect of the electric field leading to further ionization events, creating a phenomenon known as an electron avalanche, which in turn maintains the plasma state. Properties of plasma that differ from those of neutral particles including conductivity, luminescence, and chemical activation are derived from the collision between electrons in the plasma and gas molecules.42
This section explains the ionization process for the atoms. When an electron e collides with atom X, the elastic collision takes place if the collision energy is small and the kinetic energy of the electrons (electron temperature) does not significantly change. Outer shell electrons may be stripped away from the atomic nucleus resulting in ionization as described in equation (2). This occurs when electrons collide with atoms with kinetic energy greater than that of the ionizing voltage. Ions with two or greater valence are sometimes generated when the electron energy is high.
An electron collision with an atom does not necessarily result in ionization. Excitation may take place where the bound electrons within the atom absorb energy during the collision and are promoted to a higher energy orbit (equation [3]). Excitation state of atom X is denoted as X*. Because the excited atom is unstable, it returns to the ground state in a very short period of time (<10-8 s). As shown in equation (4), excess energy is released at this point as photon energy hν. A collision in which excitation takes place during the first electron collision and ionization takes place during the second electron collision is referred to as a cumulative collision, expressed by equations (5) and (6). When the energy of the ordered stable state X* of atom X is higher than the ionization voltage of atom Y, atom Y ionizes through its collision with X*. This phenomenon is known as the Penning effect in which atom Y can be ionized at almost 100% probability resulting in highly effective ionization, leading to efficient generation of plasma. Although ionization may also occur via collision of ions (equation [8]), collision of neutral particles (equation [9]), or by interaction with light (equation [10]), these processes are less likely to occur than ionization by collision of electrons and, as such, can effectively be ignored in normal weak ionization plasma.









Another process occurs in which positive ions and electrons collide and recouple back into neutral particles. Recoupling of positive ions and electrons occur via a three-body collision of positive ion-electron-electron (equation [11]) and positive ion-electron-neutral particles (equation [12]). If the electrons have a high temperature, the main process is radiating recoupling in which excess energy is released as light (equation [13]).



Next, the process of collision between a molecule and an electron is explained. A process similar to the collision of an atom and an electron occurs, and molecule XY may be ionized directly through collision with an electron (equation [14]) or become excited (equation [15]). The colliding particles may result in promotion of electrons in an atom
to a higher energy orbit. If the electron gains sufficient energy to overcome the Coulombic force that maintains its association with the positively charged nucleus, the electron is ejected and the atom ionizes to a positively charged ion. Molecules in an excited state where the total sum of internal energy of the particle changed before and after an inelastic collision is called an excited molecule, denoted by the symbol XY*. An electron shifted to the orbit of an excited state will subsequently drop back to the ground state after a short period of time, and this excess energy is released as a photon resulting in a glowing plasma.
to a higher energy orbit. If the electron gains sufficient energy to overcome the Coulombic force that maintains its association with the positively charged nucleus, the electron is ejected and the atom ionizes to a positively charged ion. Molecules in an excited state where the total sum of internal energy of the particle changed before and after an inelastic collision is called an excited molecule, denoted by the symbol XY*. An electron shifted to the orbit of an excited state will subsequently drop back to the ground state after a short period of time, and this excess energy is released as a photon resulting in a glowing plasma.
A dissociation process in which a molecule dissociates into several molecules and atoms by collision with an electron may also occur. Through collision with an electron, molecule XY dissociates into X and Y, generating radicals with unpaired electrons. Neutral radicals generated during the dissociation process, as shown in equations (16), (17), and (18), increase the chemical reactivity of the plasma. Dissociation into X and Y by electron collision does not take place at the ground state but occurs spontaneously after the molecule is excited by collision with electrons. When an electron of low energy collides with a molecule, the molecule transiently becomes an anion (equation [19]) with an electron dissociating from the molecule (equation [20]).








Atmospheric gas is a very good insulator that does not conduct electricity.45 As an insulator, gas ionizes and generates plasma when a high voltage is applied between an anode and a cathode. At this point, a dielectric breakdown (discharge) takes place when the conductivity of the gas rises rapidly. Dielectric breakdown occurs when accidental electrons that exist between electrodes serve as an initiator for the generation of plasma. Accidental electrons accelerated by the work of the electric field cause ionization as they collide with neutral molecules and atoms between the electrodes. Electrons produced through this ionization process are also accelerated by the electric field, causing further ionization. The chain of events increases the density of charged particles between the electrodes, eventually leading to a dielectric breakdown in the gas. This ionization phenomenon in the gas phase is described by Townsend theory.46
According to the Townsend theory, weak current (undercurrent) I prior to the start of a discharge is proportional to the initial electron current I0 and increases exponentially with the distance between electrodes d.

If we suppose the number of collisions and ionizations caused over a unit length by a single electron under the force of an electric field is called α (first Townsend coefficient, otherwise known as α coefficient), this collision-ionization process is referred to as α action. The α coefficient depends on pressure p and electric field
and can be expressed by the following equation. A and B are constants determined by the type of gas between the electrodes.


These equations describe a process known as an electron avalanche, in which initial electrons that serve as a trigger to the start of the discharge are accelerated by an electric field, collide, and ionize neutral particles. The number of electrons then multiplies in a manner reminiscent to that of an avalanche. An increase in current I depends on the presence of the initial electron current I0. In the absence of I0, the electron avalanche stops and exponential growth of charged particles does not occur, and a plasma is not generated.
Positive ions generated in a pair with electrons by α action accelerate toward the cathode by an electric field and collide with the cathode. Given that secondary electrons are released from a surface of a solid when high-energy ions and photons collide with the solid, Townsend named this release of secondary electrons from the cathode surface as γ action and the number of electrons released per colliding positive ion as γ coefficient. Note that β action is a phenomenon in which ionization takes place as ions collide with gas molecules, but, in reality, ion energy is insufficient to cause ionization. We calculate the discharged current that flows to the electrodes by taking α and γ actions into account. An increase in electron current is expressed as I0exp(αd) – I0, and assuming a monovalent positive ion was generated from ionization by α action, then the increase in electron current is equal to the number of positive ions. The positive ions are also influenced by the electric field but, in this case, accelerate in the cathode direction, releasing secondary electrons by γ action upon collision with the cathode. The secondary electron current at the cathode is γI0{exp(αd) – 1}.
Secondary electrons released by γ action are accelerated in the anode direction by the electric field as was the case for the initial electrons, and collisions and ionization events take place due to α action. When the secondary electron reaches the anode, electron current increases to γI0{exp(αd) – 1}exp(αd). The α and γ actions are then repeated through this process. Addition of all the electron current that reaches the anode yields the following equation.
Secondary electrons released by γ action are accelerated in the anode direction by the electric field as was the case for the initial electrons, and collisions and ionization events take place due to α action. When the secondary electron reaches the anode, electron current increases to γI0{exp(αd) – 1}exp(αd). The α and γ actions are then repeated through this process. Addition of all the electron current that reaches the anode yields the following equation.

When the denominator of equation 23 is zero, I becomes infinite and shows an electrically shorted state. Therefore, plasma is generated between the electrodes and a breakdown has taken place. In light of the earlier discussion, Townsend expressed the condition for the start of the discharge as follows, which is called the Townsend breakdown criterion.

With regard to the relationship between the voltage and the start of a discharge (spark voltage) and gas pressure (p) as well as the length of the gap between electrodes (d), this voltage is determined by the product of p and d and has a minimum value. This relationship is known as Paschen law, and a graph of the relationship between voltage at the start of discharge Vs and the product of p and d (or pd) is known as Paschen curve.
Experimentally derived Paschen law can also be derived theoretically from Townsend spark condition equation (24). Equation (24) is rewritten as ad equation as follows.


where voltage between electrodes Ed is equal to voltage Vs at the start of the discharge, and calculating spark voltage from equations (22) and (26) yields:

where C is the constant determined by the gas and cathode material. From equation (27), small product of pd results in a greater value of voltage Vs at the start of discharge, but as the product of pd becomes greater, Vs gradually increases after reaching a minimum value.

Depending on the maintenance mechanism of plasma, forms of plasma are categorized into arc discharge, corona discharge, and glow discharge. As for the method of generating plasma, its categorization includes those that depend on the frequency of the power source, such as direct current discharge and high-frequency discharge; those that depend on ambient gas pressure, such as low-pressure and atmospheric pressure plasma; and those that depend on the electrode shape and form of discharge.4,47 Among them, for the purposes of medical and bio-applications, several discharge methods for generating plasmas have been used including corona, glow, dielectric barrier, pulse, and high frequency. This section focuses on plasma generation methods that are most frequently used in plasma medical and bio-applications.
Corona Discharge
A corona discharge occurs when an electric field is concentrated at the tip of a needle. The electrodes are structured so that the electric field surrounding the electrode is uneven (such as a combination of a needle and a plate electrode) and a voltage is then applied. At this point, neutral gas near the tip of the needle ionizes by the concentrated electric field and generates plasma. A discharge caused by a local dielectric breakdown through concentration of the electric field is called a corona discharge. As discussed next, there are several modes of corona discharge. The tip of the needle electrode glows weakly as a result of a current running between electrodes at a few microamperes, forming a state of plasma known as glow corona. Raising the voltage further, the plasma at the needle tip extends along the plate electrode and becomes a state known as brush corona generated from an electric current with a pulse of approximately 1 µs. Raising the voltage even further, the plasma extended from the tip of the needle electrode connects to the plate electrode, forming a stringlike state known as streamer corona. Streamer corona is generated in a high-pressure region (700 Pa · m), and its mechanism of formation cannot be explained by the Townsend theory. This discharge phenomenon occurs over a very short period of time of less than 10-7 seconds and is not influenced by ions. As such, the discharge does not require γ action. In the streamer theory, α action on initial electrons causes an electron avalanche, and its intense spatially charged electric field accelerates electrons formed by photoionization and creates a smaller electron avalanche. A streamer is generated when this secondary electron avalanche enters the plasma pillar formed by the primary avalanche.
Glow Discharge
Glow discharge is a sustainable discharge phenomenon that typically occurs when 100 V to several kV of direct voltage is applied to a gas with a low pressure of approximately 100 Pa. Plasma generated by glow discharge is a nonthermal equilibrium plasma where energy of electrons is greater than the ion energy. The ability of the plasma generated by glow discharge to process at a low temperature gives it a wide range of potential applications. Atmospheric glow discharge can generate plasma under atmospheric pressure, leading to the generation of plasma using various gases such as He and Ar, air, and N2. Compared to other forms of discharge, glow discharge is spatially highly homogeneous and is capable of generating plasma at low temperature and in a large volume. These properties make the plasma useful for applications in the field of medicine and the biosciences. An example of a discharge plasma is an atmospheric pressure plasma jet, which uses a mixture of He and Ar gas. Atmospheric pressure plasma jet is generally obtained by sending gas into an insulator tube of several millimeters in diameter and applying a high voltage of several kilovolts to several tens of kilovolts to the tube. A typical use is of an afterglow discharge type in which high-temperature plasma generated inside the tube, made of either glass or ceramic, is pushed out of the tube as it is cooled.
Dielectric Barrier Discharge
Barrier discharge is a form of discharge that is used frequently because it can be generated relatively easily under atmospheric pressure. This approach generates streamer discharge over a large area by placing a dielectric that does not conduct electricity on either side or both sides of electrodes to prevent arc discharge and by applying an alternating high voltage ranging from 10 Hz to several kilohertz. The temperature of the ions and neutral particles does not increase because the discharge takes place over a very short period of time. Aside from being called a dielectric barrier discharge (DBD), the discharge is also known as a silent discharge. The gap between the electrodes is the plasma-processing region, known as the gap length, that is generally in the range of several millimeters to several centimeters, which is quite narrow. To improve on the arrangement, another type of discharge, known as floating electrode DBD (FE-DBD), has been developed where one of the electrodes is replaced with a floating electrode.
Pulse Discharge
Although the method of generating plasma by atmospheric discharge is very attractive for applications in biological and medical sciences, it nonetheless transitions easily into an arc discharge, and the generation of a stable plasma in a large area is difficult to achieve. By applying voltages in the form of short pulses, electrons are accelerated but heavy ions are not, creating a stable discharge even under atmospheric pressure. Because voltages with a very short duration are applied, the transition into an arc discharge can also be suppressed. With the development of magnetic pulse compression circuit technology, a short-pulsed, highly repetitive, small-scale, high-voltage power source may be feasible.
High-Frequency Plasma
This plasma is typically generated with radiofrequency (RF) electromagnetic radiation of 13.56 MHz. Electrodes are not necessarily needed, and plasma can be generated using antennas. Highly dense plasma with a large volume can be generated under low atmospheric pressure, and its low temperature tends to prevent objects from being damaged. Discharge can be maintained without secondary electron emission (γ action) from the cathode. Depending on the shape of the antenna, this plasma is classified into capacitively coupled plasma (CCP) and inductively coupled plasma (ICP).

Plasma has been shown to display broad-spectrum microbicidal activity as well as the ability to eliminate toxins associated with microorganisms.17,48,49 As such, plasma is a useful means of disinfection/sterilization. In the next section, we introduce current knowledge on the inactivation of microorganisms by plasma, together with an introduction to the authors’ own studies in this field. Generally, microbes exhibit a wide variation in intrinsic resistance to disinfectants (Figure 34.3). To date, plasma disinfection covers almost all the microbiological hierarchy from the most susceptible, enveloped viruses, to the most resistant pathogens, prions.
Bacteria
Recently, extensive research has been performed on the use of plasma to inactivate various bacteria. Escherichia coli, Bacillus species, Salmonella species, Staphylococcus aureus, and Enterococcus faecalis, in addition to bacterial spores such as G stearothermophilus, have been shown to be efficiently inactivated by plasma treatment,50,51,52,53 indicating that this technology is highly effective as a means of disinfection.
There are numerous recent reviews covering a range of different topics concerned with disinfection/sterilization using plasma. Moisan et al54 describes inactivation of several bacterial species including E coli, S aureus, and Bacillus
subtilis spores using discharge of microwaves and DBD and various gases (air; Ar; O2; CO2; and mixtures of N2, O2, and Ar), which are compared and discussed. Ehlbeck et al55 summarizes the inactivation efficiency of various plasma sources including discharge of corona, microwaves, as well as DBD and plasma jet against about 20 different microorganisms including gram-positive and gram-negative bacteria. A comparison of the inactivation of various bacteria was performed by Scholtz et al.56,57 These studies showed that the sensitivity of all vegetative bacteria was comparable, whereas Candida and Geobacillus spores56 or yeast57 are more resistant to plasma than vegetative bacteria.
subtilis spores using discharge of microwaves and DBD and various gases (air; Ar; O2; CO2; and mixtures of N2, O2, and Ar), which are compared and discussed. Ehlbeck et al55 summarizes the inactivation efficiency of various plasma sources including discharge of corona, microwaves, as well as DBD and plasma jet against about 20 different microorganisms including gram-positive and gram-negative bacteria. A comparison of the inactivation of various bacteria was performed by Scholtz et al.56,57 These studies showed that the sensitivity of all vegetative bacteria was comparable, whereas Candida and Geobacillus spores56 or yeast57 are more resistant to plasma than vegetative bacteria.
A most promising application of plasma disinfection is the eradication of antibiotic-resistant bacteria. Recently, the growing risk of disease from drug-resistant pathogens has caused considerable public health concern. Indeed, the danger of antibiotic-resistant bacteria was recently prioritized by both the World Health Organization (WHO)58 and the Centers for Disease Control and Prevention (CDC).59 The extensive and indiscriminate use of antibiotics has been recognized to alter the environmental microbiome, contributing to the emergence of drug-resistant bacteria.60 Thus, innovative methods for inactivating multidrug-resistant bacteria are sought. The plasma method is especially useful for eliminating multidrug-resistant bacteria that are otherwise difficult to neutralize.61,62 This is because the mechanisms of action of the plasma are unlikely to differ between multidrug-resistant and susceptible bacteria.
Nonetheless, there is a paucity of published studies devoted to identifying potential mechanisms of plasma-induced bacterial inactivation. Our research group analyzed the bactericidal/disinfecting effect of N2 gas plasma on Salmonella and its influence on components of the bacterial cell as well as which factors constitute the main mechanism of disinfection by N2 gas plasma from a BLP-TES (Bi-polar and Low-pressure Plasma-Triple Effects Sterilization) device,63 where a fast high-voltage pulse applied by a static induction (SI) thyristor power supply was used for plasma generation (see Figure 34.2). Our biochemical analyses demonstrated that Salmonella exposed to N2 gas plasma have altered cell surface O-antigens and DNA, whereas the plasma treatment did not influence the lipopolysaccharide (LPS) component located in the inner region of the cell wall (Figure 34.4). The lack of LPS changes may be due to low penetration of the plasma. Images obtained from scanning electron microscope (SEM) highlighted changes at the bacterial cell surface. These alterations to the outer region of Salmonella may be related to the mechanisms
by which N2 gas plasma inactivates the bacteria. However, further biochemical analyses of cell components using both gram-negative and gram-positive bacteria as well as alternative plasma sources are needed to fully elucidate whether the mechanism of inactivation is common among various bacterial species and different forms of plasma.
by which N2 gas plasma inactivates the bacteria. However, further biochemical analyses of cell components using both gram-negative and gram-positive bacteria as well as alternative plasma sources are needed to fully elucidate whether the mechanism of inactivation is common among various bacterial species and different forms of plasma.
![]() FIGURE 34.4 Nitrogen gas plasma treatment induces biochemical changes in Salmonella. A, After nitrogen gas plasma treatment with BLP-TES device, the Salmonella samples were subjected to immunochromatography for the O-antigen (Singlepath Salmonella; Merck Group, Darmstadt, Germany). The bands for Salmonella O-antigen are indicated by an arrowhead. C, control line; T, test line. B, The plasma-treated Salmonella samples were subjected to the chromogenic Limulus test for lipopolysaccharide (LPS) (Limulus-Color KY Series; Wako Pure Chemical Industries, Ltd, Osaka, Japan). The LPS concentration is shown in terms of endotoxin units per milliliter (EU/mL). C, The plasmatreated Salmonella samples were subjected to polymerase chain reaction (PCR) analysis using Salmonella One-Shot PCR (Takara Bio Inc, Kusatsu, Japan) for the invA gene. Negative control (-), which lacks DNA, is also shown. D, Real-time PCR assay by CycleavePCR Salmonella Detection Kit Ver. 2.0 (Takara Bio Inc, Kusatsu, Japan) using nitrogen gas plasma-treated Salmonella. Differences where P <.05 (asterisk) and P <.01 (double asterisks) versus control (0 min) were considered significant. For a color version of this art, please consult the eBook. Reprinted from Maeda et al.63 Copyright © 2014 Elsevier. With permission. |
Bacterial Spores
Except for prions, bacterial spores are generally considered to be the most resistant microorganisms to inactivate. Indeed, bacterial spores are often used as an index for the demonstration of sterilization effectiveness.64 By contrast, vegetative bacteria are generally susceptible to disinfection/sterilization treatment.65 Next, we introduce a selection of representative papers related to inactivation of bacterial spores by plasma.
Lerouge et al66 used low-temperature plasma generated from several different gases to inactivate B subtilis spores. Potential etching of spores upon treatment with various gas plasmas was compared to that induced by O2 gas plasmas. Plasmas of Ar, hydrogen (H2), CO2, and tetrafluoromethane (CF4) gases did not present any etching phenomenon. Etching resistance was discussed in terms of spore morphology and spore complex coating structure. The D-value of O2/CF4
was 1.5 minutes, whereas that of pure O2 was 3.8 minutes. These observations indicate O2/CF4 was more efficient at spore inactivation with reduced levels of etching, which enhances functional/material compatibility while maintaining a sterility assurance level (SAL) of 10-6.
was 1.5 minutes, whereas that of pure O2 was 3.8 minutes. These observations indicate O2/CF4 was more efficient at spore inactivation with reduced levels of etching, which enhances functional/material compatibility while maintaining a sterility assurance level (SAL) of 10-6.
Lassen et al67 optimized the inactivation of G stearothermophilus ATCC 7953 endospores using RF-generated CF4/O2 gas plasma in terms of several factors such as power, flow rate, exposure time, and RF system type. Specifically, the dependency of the sporicidal effect on spores inoculated in the chamber of the RF system was studied based on survival curves and D-values. Their results indicated that the power value of the RF excitation source was the only parameter to influence inactivation of endospore.
Lassen et al68 also evaluated the sporicidal effect of different RF plasma processes produced by combining various gas mixtures. Sporicidal effects of O2-containing gas plasma were found to be dependent on the power. In the absence of O2, no power dependency was observed. Survival curves obtained with the use of plasma generated from Ar/H2 indicated a straight line from the initial population that may be used to extrapolate on SAL of 10-6 and less etching. The CF4/O2 caused more damage to the material by etching than Ar/H2, indicating that the sporicidal and etching phenomenon do not always coincide. The CF4/O2 gas mixture may be appropriate for inactivation efficiency but inappropriate in attaining functional and material compatibility due to etching by O2 gas. Inactivation through etching by O2 gas was a highly power-dependent phenomenon. The authors concluded that an inactivation mechanism might combine a sporicidal effect with substrate damage by etching of the endospore with O2 gas, whereas the effect of the presence of ultraviolet (UV) radiation is negligible.
Hury et al69 studied the inactivation of Bacillus spores in O2-based plasma. The study clearly shows O2, H2O2, and CO2 plasmas to be more efficient in eliminating B subtilis spores than Ar plasma.
Takamatsu et al24 suggest the reactive species responsible for the inactivation of endospores in CO2 plasma is singlet O2 (1O2) and that of N2 gas plasma is the hydroxyl (•OH) radical. Variation in bacterial density on the carrier material leads to significant differences in the inactivation efficiency of spores when using CO2 plasma. This observation is due to the shallow penetration depth of reactive species of CO2 gas plasma (1O2, 10-40 nm). If the vegetative cells form multiple layers, such as in a biofilm, the killing efficiency is drastically reduced.
Winter et al70 reported the Ar gas plasma inactivation of B subtilis endospores. The authors investigated the plasma-specific response of B subtilis toward Ar as well as air-generated plasmas. Cellular responses, such as DNA damage and oxidative stress, were observed due to the synergistic effect of Ar and air plasma treatment. A variety of gas-dependent cellular responses such as growth retardation and morphologic changes, including shrinkage by O2 gas in air, were also observed.
Fungi
Compared to vegetative bacteria, studies on plasma-treated fungi are limited. Nonetheless, it is clear that fungi are more resistant to plasma than vegetative bacteria because they require a longer exposure time to achieve an equivalent level of inactivation. We showed that N2 gas plasma generated from a BLP-TES device inactivated Aspergillus brasiliensis, which belongs to the same genus as the aflatoxin B1 (AFB1)-producing fungi.21 However, the viable cell number of A brasiliensis was unchanged after 5-minute treatment,21 whereas Salmonella was completely inactivated after an identical exposure time.63 Nonetheless, the viable cell count of Aspergillus significantly decreased following treatment with N2 gas plasma for 15 minutes or more. Therefore, the N2 gas plasma treatment inactivates molds but needs a longer time for inactivation compared to bacteria.
Similarly, Soušková et al reported no significant differences in susceptibility against plasma generated by corona discharge among bacteria including E coli and Staphylococcus epidermidis, but the susceptibility of fungi is different between species including Aspergillus oryzae, Cladosporium sphaerospermum, and Penicillium crustosum.71,72,73 Intriguingly, of these fungi, Aspergillus displayed the greatest resistance to plasma inactivation.
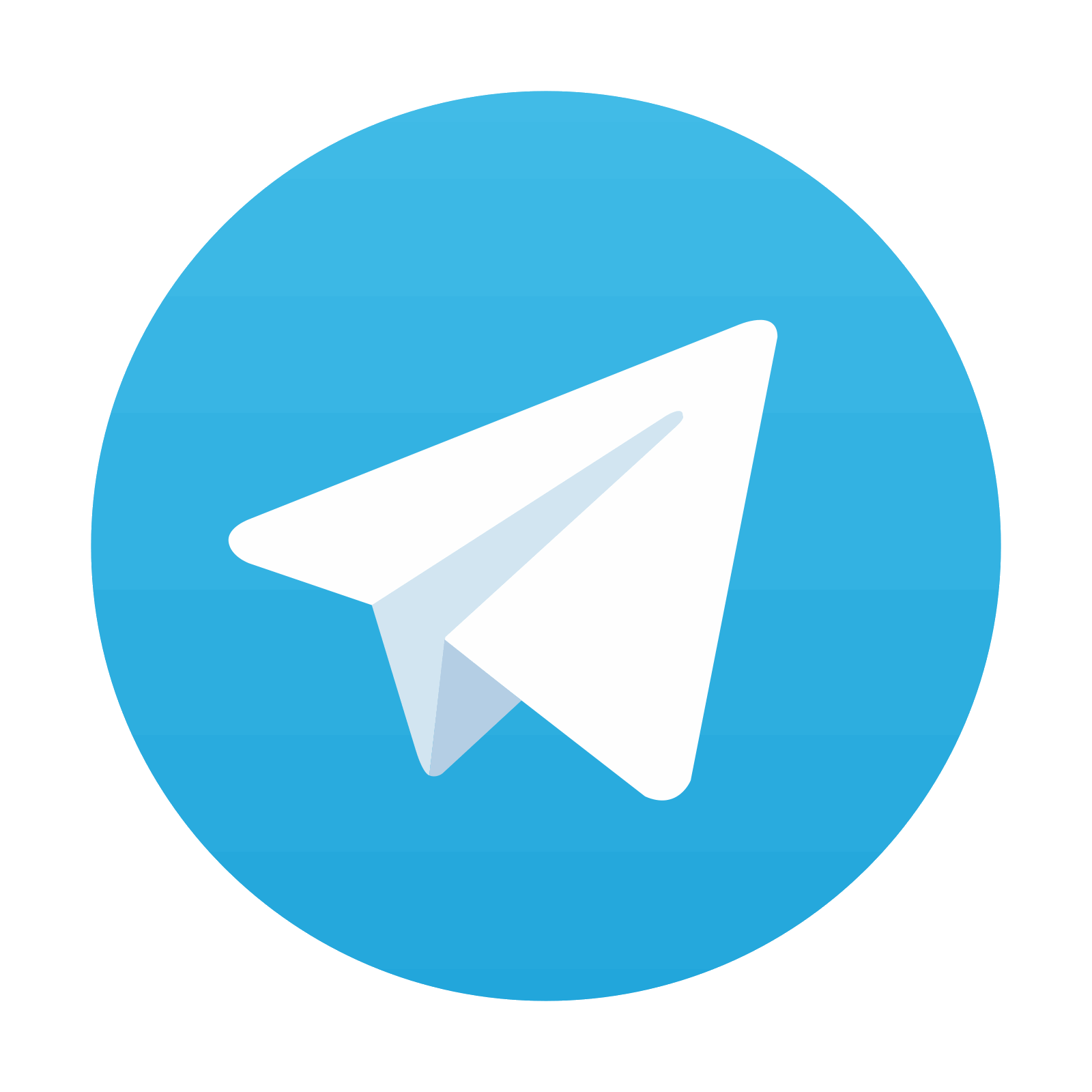
Stay updated, free articles. Join our Telegram channel
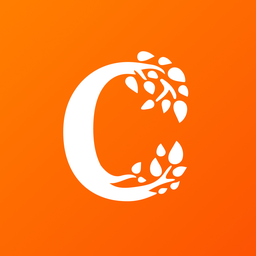
Full access? Get Clinical Tree
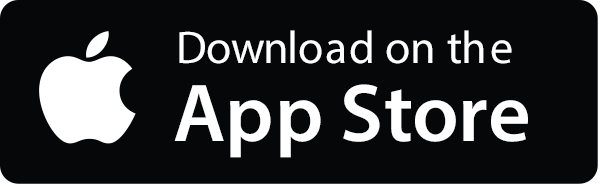
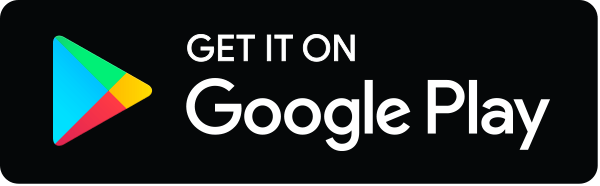