Chapter 13 Plasma proteins and enzymes
Introduction
Proteins are present in all body fluids, but it is the proteins of the blood plasma that are examined most frequently for diagnostic purposes. Over 100 individual proteins have a physiological function in the plasma. Their principal functions, and some of the proteins, are indicated in Figure 13.1. Quantitatively, the single most important protein is albumin. With the exception of fibrinogen, the other proteins are known collectively as globulins. Changes in the concentrations of individual proteins occur in many conditions and their measurement can provide useful diagnostic information.
Measurement of plasma proteins
Total plasma protein
The total protein concentration of plasma can also fall rapidly if capillary permeability increases, because protein will diffuse out into the interstitial space. This can be seen, for example, in patients with septicaemia or generalized inflammatory conditions. Causes of increased and decreased total plasma protein concentration are summarized in Figure 13.2.
Protein electrophoresis
Electrophoresis, on cellulose acetate or agarose gel, separates the proteins into distinct bands: albumin, α1-and α2–globulins, β-globulins and γ-globulins. Plasma proteins are often still classified into groups according to their electrophoretic mobility (Fig. 13.3), although this classification has no relevance in relation to their function, with the exception that the normal immunoglobulins migrate (and are still often referred to) as γ-globulins.
Figure 13.4 shows diagrammatically the appearance of normal serum (A) and serum containing a paraprotein (B) after electrophoresis on agarose gel and staining with a protein-sensitive stain. Note that in (B) there is a decrease in normal immunoglobulins, as characteristically occurs in patients with paraproteinaemia due to myeloma (see p. 231). Paraproteins typically migrate in the γ-region but (especially the IgA class) may migrate more anodally, towards the β region. Pattern (C) shows a polyclonal increase in immunoglobulins (as may occur in some autoimmune diseases and chronic infections).
Specific plasma proteins
Albumin
In hypoalbuminaemic states, the decreased plasma oncotic pressure disturbs the equilibrium between plasma and interstitial fluid, with the result that there is a decrease in the movement of the interstitial fluid back into the blood at the venular end of the capillaries (Fig. 13.5). The accumulation of interstitial fluid is seen clinically as oedema. The relative decrease in plasma volume results in a fall in renal blood flow. This stimulates the secretion of renin, and hence of aldosterone, through the formation of angiotensin (secondary aldosteronism, see p. 24). This results in sodium retention and thus an increase in ECF volume, which potentiates the oedema.
There are many possible causes of hypoalbuminaemia (Fig. 13.6), a combination of which may be implicated in individual cases. For example, in a patient with malabsorption due to Crohn’s disease, a low albumin may reflect both decreased synthesis (decreased supply of amino acids due to malabsorption) and increased loss (directly into the gut from ulcerated mucosa).
Measurements of albumin concentration are frequently used in relation to the provision of nutritional support. This topic is discussed in detail in Chapter 20, but it should be noted that, because of its relatively long plasma half-life (approximately 20 days), plasma albumin concentration is not a useful marker of the response to nutritional support in the short term (<10 days).
Albumin is a high-capacity, low-affinity transport protein for many substances, such as thyroid hormones, calcium and fatty acids. The influence of low plasma albumin concentration on measurements of thyroid hormones and calcium is considered on pp. 154 and 209, respectively. Albumin binds unconjugated bilirubin, and hypoalbuminaemia increases the risk of kernicterus in infants with unconjugated hyperbilirubinaemia. Salicylates, which displace bilirubin from albumin, can have a similar effect.
Caeruloplasmin
This is a copper-carrying protein, which functions as a ferroxidase and superoxide scavenger. Its synthesis and plasma concentration are greatly reduced in Wilson’s disease (p. 100). Its concentration is increased in pregnancy (an oestrogen-related effect). It is an acute phase protein.
Transferrin
This β-globulin is the major iron-transporting protein in the plasma; normally about 30% saturated with iron, it is characteristically more than 60% saturated in haemochromatosis. Its measurement as an index of nutritional status is discussed in Chapter 20. Transferrin and ferritin are discussed in more detail in Chapter 17. Ferritin is also an iron-carrying protein, and measurement of its plasma concentration is used as a test for assessing body iron stores. The plasma concentration of carbohydrate-deficient transferrin can be used to assess exposure to alcohol (see p. 95), although it is neither a specific nor a sensitive test.
Other plasma proteins
Measurements of other plasma proteins may provide useful information in particular circumstances. Measurement of coagulation factors (fibrinogen, factor VIII and others) is usually carried out in haematology laboratories and is essential in the investigation of some bleeding disorders. Measurement of the proteins of the complement system is of considerable value in the investigation of some diseases with an immunological basis. The apolipoproteins are considered in detail in Chapter 14. The importance of hormone-binding proteins, such as cortisol-binding globulin and sex hormone-binding globulin, is considered in Chapters 8 and 10, respectively. Plasma proteins used in the assessment of nutritional status are discussed in Chapter 20. Measurement of the plasma concentration of β2–microglobulin is of value in monitoring patients with myeloma (see p. 232). The measurement of plasma proteins derived from tumours (tumour markers) is discussed in Chapter 18.
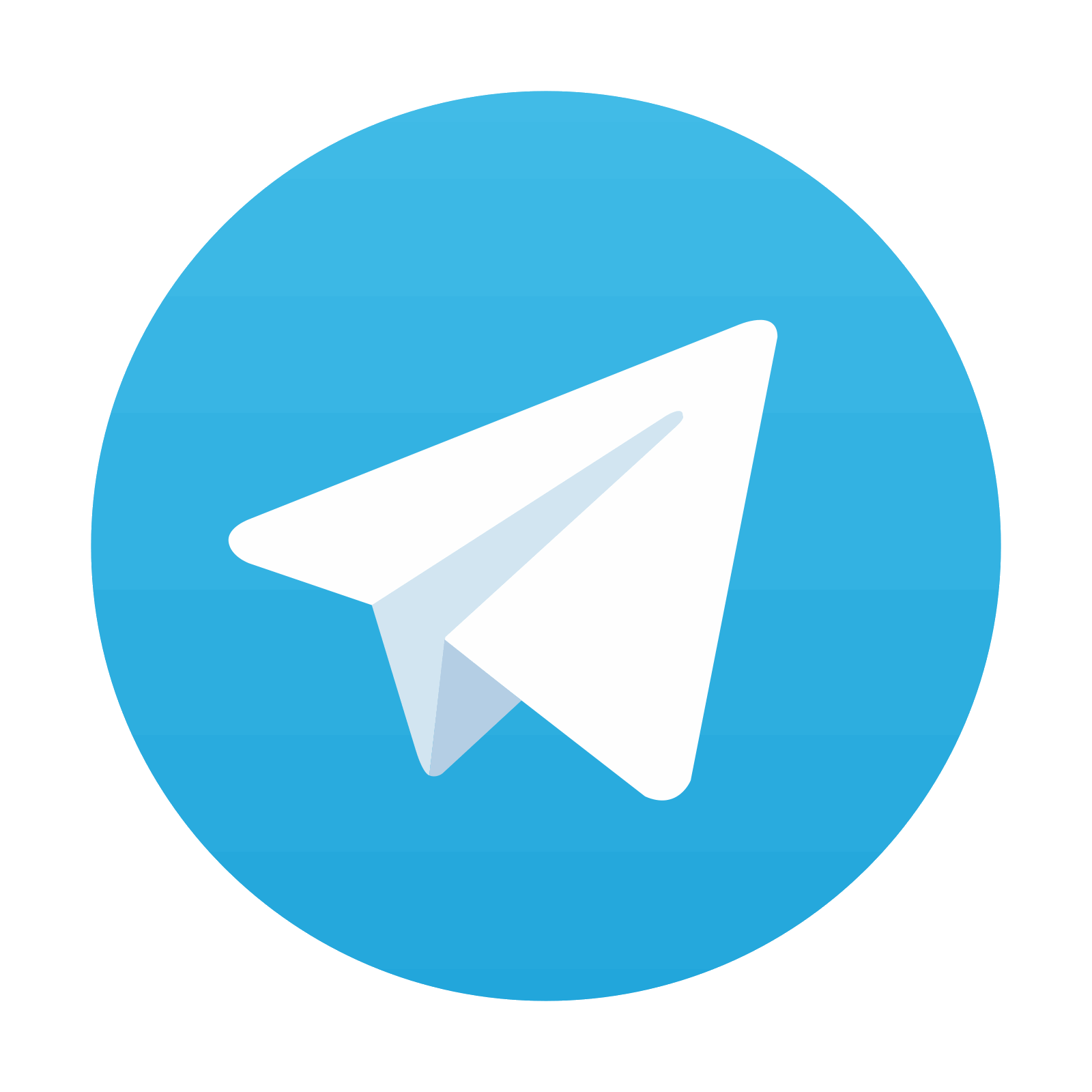
Stay updated, free articles. Join our Telegram channel
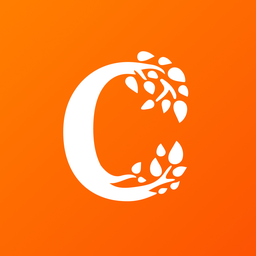
Full access? Get Clinical Tree
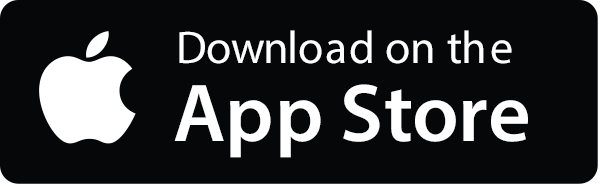
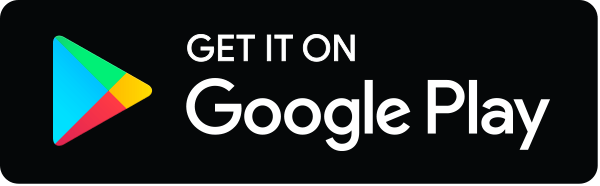