(1)
Department of Chemistry, KU Leuven, Leuven, Belgium
Abstract
In the last two decades, fluorescent proteins became an indispensable tool to noninvasively label a protein in living cells. The discovery of photoswitchable fluorescent proteins expanded the applications of the fluorescent proteins to techniques such as molecular tracking and highlighting on a microscope. Recently, a new microscopic modality to achieve a superresolution circumventing the diffraction limit of light (photoactivated localization microscopy, PALM) has been developed based on the photoswitchable fluorescent proteins. Here we introduce a basic protocol of PALM through the visualization of actin bundles with superresolution.
Key words
Actin bundlesDiffraction limitFluorescent proteinPhotoswitchable fluorescent proteinSuperresolution microscopyPALM1 Introduction
Fluorescent labeling of molecules is crucial for imaging by fluorescence microscopy. Despite a wide variety of organic fluorophores available to label molecules in vitro, labeling of intracellular molecules under living conditions remains difficult. The gene encoding green fluorescent protein (GFP) was cloned from Aequorea victoria in 1992 [1]. GFP and GFP-like fluorescent proteins form a chromophore self-catalytically from its own amino acid residues without any cofactors. Capability of fluorescence emission upon ectopic expression of the gene encoding GFP was shown in 1994 in prokaryotic and eukaryotic cells [2]. These findings provided a way to label proteins in living cells noninvasively by genetic engineering with GFP. A lot of efforts have been made to produce fluorescent proteins with different photophysical properties, such as color variants and brighter fluorescence by mutagenesis and cloning of novel proteins from different sea organisms (for review, see [3–7]). On the way, we encountered a series of interesting fluorescent proteins whose fluorescence color/intensity can be regulated upon light illumination, so-called photoswitchable fluorescent proteins [8, 9]. The photoswitchable fluorescent proteins give rise to a new method to track molecules. For example, nucleocytoplasmic shuttling of extracellular signal-regulated kinase 1 (ERK1) has been observed by expressing ERK1 fused to a photoswitchable fluorescent protein, Dronpa [9]. This experiment revealed transient bidirectional acceleration of ERK1-Dronpa transport across the plasma membrane upon epidermal growth factor stimulation. Molecular highlighting is another example of an application. By using hippocampal neuron primary culture expressing a photoswitchable fluorescent protein, Kaede, a neuron in the culture can be highlighted with different color upon UV illumination to the specific cell [8, 10]. This method is also applicable to an in vivo system; Sato et al. developed a transgenic zebrafish expressing Kaede in neuronal cells as a tool to label neural morphology [11]. Application of the photoswitchable fluorescent proteins as proteinous media for data storage has also been proposed [12, 13].
So far three classes of photoswitchable fluorescent proteins have been reported: photoactivatable (PAFP), photoconvertible (PCFP), and reversibly switchable (RSFP) (Table 1, Fig. 1). PAFPs are originally nonfluorescent and become fluorescent upon light illumination. The first PAFP, PAGFP, was made by introducing a mutation T203H to wild-type Aequorea GFP [14]. PAGFP achieves green fluorescence upon UV illumination. The photoactivation of PAGFP is thought to be the result of decarboxylation from the side chain of E222, which is placed nearby the chromophore, followed by change in the local environment around the chromophore and shift of the chromophore from nonfluorescent protonated to fluorescent anionic form (Fig. 2) [15]. Photoactivatable fluorescent proteins in the red range, PAmRFP1, PAmCherry, and PATagRFP, have also been engineered based on red fluorescent proteins [16–18].
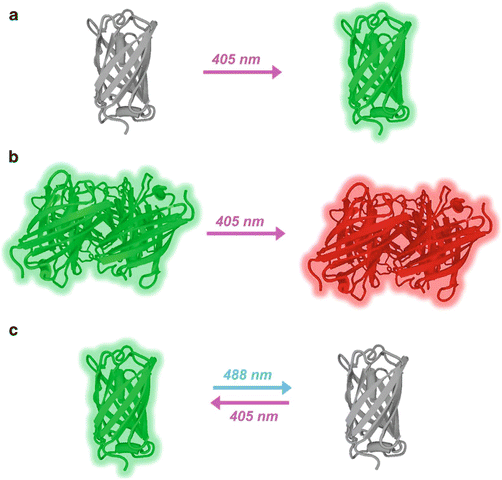
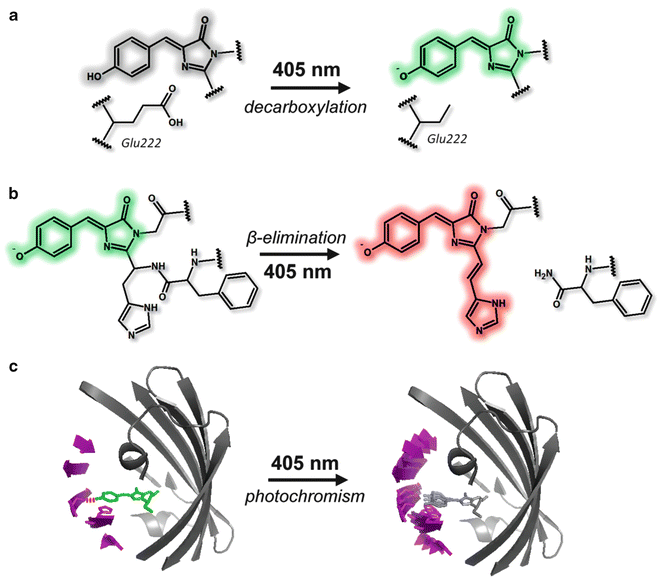
Table 1
Selected photoswitchable fluorescent proteins
Protein | Quaternary structurea | Laser line for excitationb | Emission maximum (nm) | Laser line for switchingb | Available from | Reference |
---|---|---|---|---|---|---|
Photoactivatable | ||||||
nf → green | ||||||
PAGFP | m | 488 | 515 | 405 | Addgene | [14] |
nf → red | ||||||
PAmRFP1 | m | 561 | 605 | 405 | Addgene | [16] |
PAmCherry1 | m | 561 | 595 | 405 | Clontech | [17] |
PATagRFP | m | 561 | 595 | 405 | Evrogen | [18] |
Photoconvertible | ||||||
Green → red | ||||||
Kaede | t | 488 (g) 561 (r) | 518 (g) 595 (r) | 405 | Amalgaam | [8] |
EosFP | t | 488 (g) 561 (r) | 516 (g) 581 (r) | 405 | MoBiTec | [19] |
tdEosFP | td | 488 (g) 561 (r) | 516 (g) 581 (r) | 405 | [19] | |
Dendra2 | m | 488 (g) 561 (r) | 507 (g) 573 (r) | 405 | Clontech | |
mEosFP | m | 488 (g) 561 (r) | 516 (g) 581 (r) | 405 | [19] | |
mEos2 | mc | 488 (g) 561 (r) | 519 (g) 584 (r) | 405 | Addgene | [23] |
mEos3.1 | m | 488 (g) 561 (r) | 519 (g) 584 (r) | 405 | [24] | |
mEos3.2 | m | 488 (g) 561 (r) | 519 (g) 584 (r) | 405 | [24] | |
KikGR | t | 488 (g) 561 (r) | 517 (g) 593 (r) | 405 | Amalgaam | [20] |
mKikGR | m | 488 (g) 561 (r) | 515 (g) 591 (r) | 405 | Amalgaam | [22] |
Cyan → green | ||||||
PSCFPd | m | 405(c) 488 (g) | 468 (c) 511 (g) | 405 | [25] | |
PSCFP2d | m | 405(c) 488 (g) | 468 (c) 511 (g) | 405 | Evrogen | |
Reversibly switchable | ||||||
![]() | ||||||
22Ge | t | 488 | 488 (off) 405 (on) | |||
Dronpa | m | 488 | 518 | 488 (off) 405 (on) | Amalgaam | [9] |
Dronpa 2 | m | 488 | 487 | 488 (off) 405 (on) | [29] | |
Dronpa 3 | m | 488 | 514 | 488 (off) 405 (on) | Amalgaam | [29] |
bsDronpa | m | 488 | 504 | 488 (off) 405 (on) | [30] | |
Padron | m | 488 | 522 | 488 (on) 405 (on) | [30] | |
rsEGFP | m | 491 | 510 | 491 (off) 405 (on) | Abberior | [13] |
Dreiklang | m | 515 | 529 | 405 (off) 369 (on) | Abberior | [33] |
![]() | ||||||
rsCherry | m | 561 | 610 | 561 (on) 445 (off) | [31] | |
rsCherryRev | m | 561 | 608 | 561 (off) 445 (on) | [31] | |
rsTagRFP | m | 561 | 585 | 561 (off) 445 (on) | [32] | |
Photoconvertible/reversibly switchable | ||||||
![]() | ||||||
IrisFP | t | 488 (g) 561 (r) | 516 (g) 580 (r) | 488(g → nf) 405 (nf → g) 405(g → r) 561 (r → nf) 440 (nf → r) | [34] | |
mIrisFP | m | 488 (g) 561 (r) | 516 (g) 578 (r) | 488(g → nf) 405 (nf → g) 405 (g → r) 561 (r → nf) 440 (nf → r) | MoBiTec | [35] |
Niji | m | 488 (g) 561 (r) | 507 (g) 569 (r) | 488(g → nf) 405 (g → r) 405(nf → g) 561 (r → nf) 440 (nf → r) | [36] |
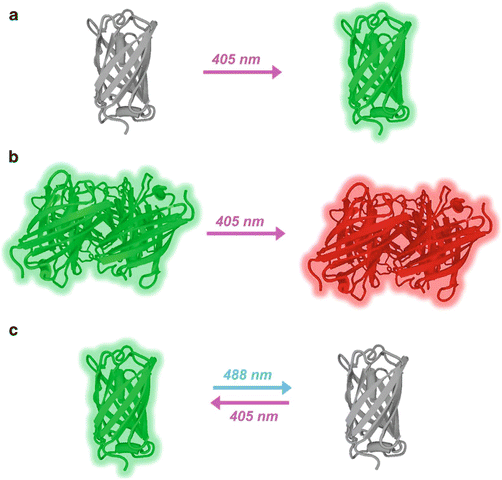
Fig. 1
Different modes of photoswitchable fluorescent proteins. (a) PAFP. PAGFP becomes fluorescent upon 405 nm illumination. (b) PCFP. Kaede originally emits green fluorescence, and the fluorescence shifts into longer wavelength (red region) upon 405 nm illumination. (c) RSFP. Dronpa originally emits green fluorescence, loses fluorescence reversibly upon 488 nm illumination, and regains upon 405 nm illumination
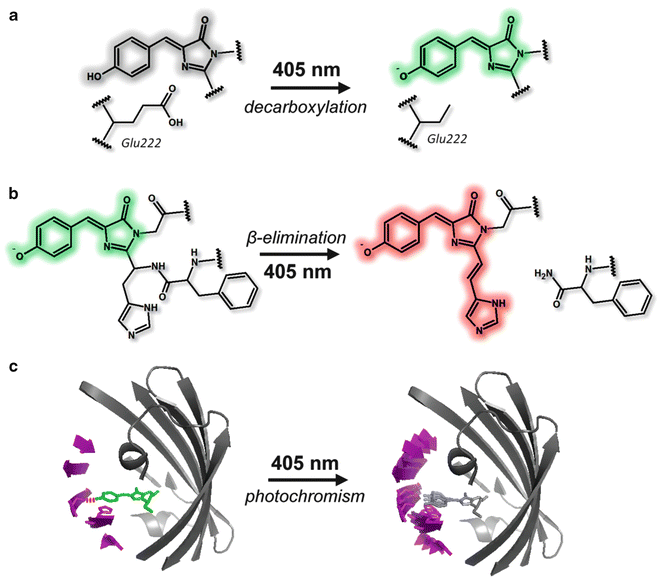
Fig. 2
Molecular mechanisms responsible for the switching behavior of photoswitchable fluorescent proteins. (a) Mechanism of photoactivation in PAGFP. The decarboxylation of Glu222 induces changes in the local environment around the chromophore and shifts the chromophore from nonfluorescent protonated to fluorescent anionic form. (b) Mechanism of the photoconversion in Kaede. UV illumination induces a formal β-elimination reaction, which results in cleavage of the main chain and produces a double bond between Cα and Cβ of His62. This double bond expands the π conjugation of the chromophore to the His62 imidazole ring and shifts the absorption and emission bands into the red region. (c) Mechanism of the reversible photoswitching of Dronpa. In the bright state, the anionic chromophore is fixed to the β-barrel via a hydrogen bond (red dotted line) and π–π stacking. In the dark state, the hydrogen bond is broken by the protonation of the chromophore, and as a result, part of the β-barrel as well as the chromophore becomes flexible. The structural flexibility in the dark state makes vibrational relaxation the dominant pathway instead of radiational relaxation
PCFPs change their fluorescent color upon light illumination. The first PCFP reported is Kaede, which was isolated from a stony coral Trachyphyllia geoffroyi [8]. Kaede originally emits green fluorescence, and upon UV illumination, the fluorescence is converted to red. The chromophore of Kaede in the green form is 4-(p-hydroxybenzylidene)-5-imidazolinone, the same as that of Aequorea GFP except the first amino acid residue of the chromophore is histidine (His62) instead of serine. UV illumination evokes a formal β-elimination reaction on His64, results in cleavage of the main chain, and produces a double bond between Cα and Cβ of His62. This double bond expands the π conjugation of the chromophore to the imidazole ring of His62 and shifts the absorption and emission bands into the red region (Fig. 2) [10]. The green-to-red conversion is irreversible, since the formal β-elimination is irreversible. Other photoconvertible fluorescent proteins, such as EosFP and KikGR, have been cloned or genetically engineered [19, 20]. A drawback for the use of these proteins is the formation of obligate homotetramer, which can induce artificial molecular interactions of the target molecule and change its behavior. To avoid this issue, monomeric mutants of the photoconvertible fluorescent proteins, such as mEosFP, Dendra2, and mKikGR, have been engineered [19, 21–24]. Most of the PCFPs show green-to-red conversion, but so far only two PCFPs that can be converted from cyan to green fluorescence, i.e., PSCFP and PSCFP2, have been engineered [25]. In this chapter, we categorized these two proteins as PCFP due to their photoswitching property, but they are often categorized into PAFP family due to their structural similarity with PAGFP.
One of the first RSFP reported is Dronpa, which was found on the way to make a monomeric mutant of tetrameric, non-switchable green fluorescent protein, 22G, isolated from Pectiniidae [9]. Dronpa originally emits green fluorescence (bright state), becomes nonfluorescent upon strong blue/green illumination (dark state), and regains fluorescence upon UV illumination. This switching is reversible. Based on X-ray crystallography, cis/trans photoisomerization of the chromophore was proposed as the molecular mechanism of the on/off transition of Dronpa [26]. Structural analysis of Dronpa by NMR gave evidence that a part of the β-barrel together with the chromophore becomes flexible only in the dark state, which provides a photochemical explanation for the absence of fluorescence in the dark state; vibrational relaxation becomes dominant in the dark state due to the flexibility (Fig. 2) [10, 27]. Since the switching is based on the photochromism of the protein, this group of proteins is sometimes referred as photochromic fluorescent protein. Several mutants of Dronpa with faster switching have been developed [28–30]. Dronpa and all its mutants show green fluorescence in the bright state. In the same spectrum range, rsEGFP has recently been engineered from Aequorea GFP [13]. There are also reversibly photoswitchable fluorescent proteins in the red range, namely, rsCherryRev and rsTagRFP [31, 32]. Most of the RSFP switch to the dark state upon the illumination at the same wavelength as the excitation and switch back to the bright state upon illumination at UV or violet range. Padron and rsCherry are exceptions. There proteins are switched to the bright state upon the illumination at the same wavelength as for excitation and converted to the dark state upon UV-violet illumination [30, 31]. In both cases the wavelength for the excitation is coupled with switching, which limits the application. Recently, an RSFP with fluorescence excitation decoupled from switching, Dreiklang, has been developed. Dreiklang emits yellow fluorescence upon excitation at 515 nm, and it is turned off and on upon illumination at 405 nm and 369 nm, respectively [33].
Some photoswitchable fluorescent proteins have both photoconvertible and reversibly switchable properties. For instance, IrisFP shows a green-to-red photoconversion upon UV illumination, and it can be turned on/off repeatedly in both green and red states [34]. IrisFP was engineered from EosFP and hence, it is tetrameric, but the monomeric version of this protein, mIrisFP, and a mutant of Dendra2 with similar properties, Niji, have been developed [35, 36].
Recently, a new superresolution fluorescence microscopic modality, photoactivated localization microscopy (PALM), has been developed based on the photoswitchable fluorescent proteins [37, 38]. In general, the highest achievable resolution of optical microscopy is determined by the diffraction limit of light. In the case of fluorescence microscopy, the best resolution is around 200 nm. PALM uses the stochastic activation of individual photoswitchable fluorophores followed by the precise determination of their position by fitting to render images with a higher resolution (Fig. 3). Weak illumination with a so-called activation laser guarantees that only a very small subset of the fluorophores that label the target molecules are in the emissive state. This subset emits sufficient photons to enable precise localization of the molecule before it enters a dark state or photobleaches. Repeating this process for multiple cycles, each generating a stochastically unique subset of molecules in the emissive state, enables the reconstruction of the image through the calculated position of each molecule. The resolution of PALM is related to the precision of the fitting, which depends on the number of photon emitted from each molecule and on the level of background. So far the achievable resolution is around 10–20 nm. In this chapter, we will introduce a basic method of PALM by using visualization of actin bundles in cultured cells with KikGR-labeled actin as an example.
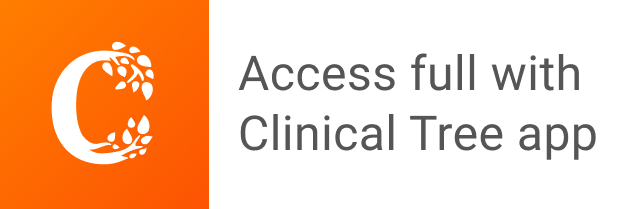