Fig. 4.1
Mammalian cell adhesion onto a biomaterial. a Initial contact between the cell and the biomaterial conditioned with serum proteins. b Bonding between cell surface receptors and protein adhesion ligands. c Cell cytoskeletal reorganization with progressive cell spreading onto the biomaterial surface. (Adapted from Ratner et al. [15])
The biological molecules involved in cell adhesion and spreading, including ECM, cell membrane and cytoskeleton proteins, interact to induce signal transduction, promote transcription factors and regulate gene expression [16, 17]. Controlling the interaction between these proteins and biomaterials surfaces is an important factor for the design of biocompatible surfaces [7, 14, 18].
Cells Involved in the Immune Response
In order to understand and predict cell-biomaterial interactions it is important to know which cells circulating in the blood are actively responding to biomaterials. Red blood cells carry hemoglobin that contains iron and is responsible for oxygen transport. White blood cells, known as leukocytes, are the ones involved in the body’s immune response. Generally, the occurrence of an infection is characterized by an increased number of leukocytes. These are grouped into granulocytes, monocytes and lymphocytes. Table 4.1 summarizes these groups of leukocytes, their abundance within the leucocytes’ population, function and relevance.
Leukocyte | Abundance*(%) | Function | Relevance |
---|---|---|---|
Granulocytes | |||
Neutrophil | 65–70 | Phagocytosis | Body defense against bacteria and other foreign substances |
Eosinophil | 3 | Allergic response | |
Basophil | <1 | Not well understood | |
Monocytes | 10 | Phagocytosis Differentiation into macrophages | Formation of foreign body giant cells |
Lymphocytes | 20 | Production and secretion of immune proteins | Immune system |
Among the granulocytes, which are the most representative group of white blood cells, the neutrophils are predominant. These cells provide a protective mechanism, as their function is to engulf and destroy foreign material.
Monocytes are also phagocytic cells capable of engulfing and destroying foreign substances. These cells change their morphology and differentiate into macrophages when going into the tissues. When the macrophage fails to phagocytize a given material, it coalesces with other cells and forms a multinucleated giant cell. Among the several types of giant cells, the Foreign Body Giant Cells (FBGCs, a collection of fused macrophages) is of major concern to biomaterials. The presence of FBGCs indicates that the phagocytic system is incapable of eliminating the foreign matter from the body. Tissue destruction often occurs and results in loss of normal tissue, loss of function of the organ, or significant bone resorption, depending on the site of inflammation [3, 19].
The lymphocytes are responsible for our immune system that protects us from disease. These cells are active protein factories and have a large nucleus that almost completely fills the cell. The small amount of cytoplasm contains the factory components for making and secreting immune proteins.
Scattered throughout the blood cells are fragments called platelets that are important in the clotting mechanisms, forming a plug to prevent excessive bleeding when the tissue is injured.
Extracellular Matrix
Normal cells respond to various environmental signals within the ECM. The interaction between cells and ECM is bidirectional and dynamic, meaning that cells are constantly accepting signals from the environment through the ECM, which in turn is often reshaped by the cells. At least three roles have been assigned to the ECM in the control of cell behavior, namely it (a) the provision of adhesion signals; (b) binding sites for growth factors; and (c) local protein breakdown for the occurrence of enzyme activity during cell migration [7, 16].
The ECM secreted by cells that populate a tissue or an organ, consists in a polymeric network of chemical and physical connections between several types of macromolecules. Its composition will be determined by factors like mechanical forces, oxygen requirements and gene expression patterns. Indeed, it is a complex mixture of structural and functional proteins (collagen and fibronectin), glycosaminoglycans (GAGs), glycoproteins and small molecules (Fig. 4.2) arranged in a unique, tissue-specific three-dimensional architecture [7, 16, 20].
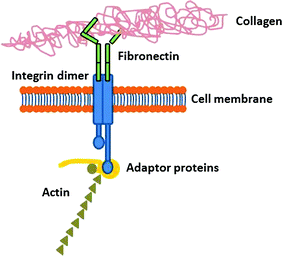
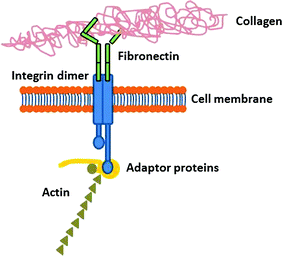
Fig. 4.2
Schematic representation of the extracellular matrix
Efforts have been conducted to mimic the ECM, i.e. by knowing its composition and how the components fit together, and how these will contribute to the advance of the biomaterials field. The rational division of the ECM into structural and functional components is not an easy task since many of these molecules present both structural and functional roles [16]. The most important ECM proteins are briefly described below.
Collagen
Collagen is the most abundant protein within the mammalian ECM, representing over 90 % of the ECM dry weight in most of the tissues and organs. More than 20 different types of collagen have been identified, each with a unique biological function [13–15]. Type I collagen is the main structural protein present in tissues. It is abundant in tendinous and ligamentous structures, and provides the necessary strength to accommodate the mechanical loading to which these tissues are commonly subjected. Furthermore, these tissues provide a convenient source of collagen for many biomedical applications. Other types of collagens can be found in the ECM in much lower amounts than Type I collagen, although providing different mechanical and physical properties to the ECM. One example is the Type IV collagen, which is present within the basement membrane of most vascular structures and tissues with an epithelial cell component [21].
Fibronectin
Fibronectin is the second most abundant protein in the ECM. It is a large dimeric glycoprotein that exists either in the soluble state or as a tissue isoform. The ECM of sub-mucosal structures, basement membranes and interstitial tissues contains great amounts of fibronectin. This protein possesses ligands for the adhesion of several cell types. Fibronectin is rich in the Arg-Gly-Asp (RGD) subunit, the most common adhesion motif, which is recognized by the cell surface receptors (integrins), thus being extremely important for cell adhesion [21]. When bound via integrins, this protein triggers a number of signal transduction pathways that activates events like cell spreading, proliferation, differentiation and migration [13]. The cell friendly features of fibronectin have made it an attractive substrate for in vitro cell culture, but also for its use to coat synthetic scaffold materials to promote biocompatibility [22].
Laminin
Laminin is an adhesion protein that is present in the ECM in numerous forms depending on the specific combination of its peptide chains. This protein is one of the most critical ECM factors in the cell and tissue differentiation [13].
Glycosaminoglycans
Depending on the tissue location, age of the host and microenvironment, several combinations of glycosaminoglycans (GAGs) can be found in the ECM. These macromolecules have various functions such as binding to growth factors and cytokines, promoting water retention and contributing to the gel properties of the matrix. Heparin and hyaluronic acid are examples of two GAGs that are present in the ECM [13].
Growth Factors
Although in small amounts, growth factors and cytokines are also present in the ECM and they act as potent cell behavior modulators. There is an extensive list of growth factors present in the ECM, including the vascular endothelial cell growth factor (VEGF), the fibroblast growth factor (FGF) family, and the epithelial cell growth factor (EGF), among others [23].
Cell Membrane Proteins—Integrins and Adhesion Proteins
The adhesion proteins are comprised of four main classes—selectins, immunoglobulin super family, adhesins and integrins—and are capable of interacting with specific ligands located at the membrane of neighbor cells or on the ECM [24]. Among the adhesion proteins, the integrins are the main cell surface receptors for proteins within the ECM [24, 25].
Integrins are transmembranar proteins that can act as a bridge between surface adsorbed ECM proteins and interacting cells. The cellular recognition of biomaterials and the progression of subsequent cellular events are essentially based on integrin-mediated interactions.
Integrins are composed of two non-covalently associated glycoprotein subunits, namely α- and β- subunits [25]. Different types of α- and β- subunits and different combinations exist, thus a large variety of integrins with the ability to bind to different types of ligands are available (Table 4.2) [26]. Many integrins are also capable of binding to more than one protein, whereas many proteins can act as ligands for more than one integrin [27, 28]. In the integrin structure, each subunit has a large extracellular domain, a transmembrane domain and a short cytoplasmic domain [25].
Table 4.2
Integrin types and respective ligands
Subunits | Ligands | |
---|---|---|
β1 | α1 | Collagens, laminins |
α2 | Collagens, laminins | |
α3 | Laminins, fibronectin, thrombospondin | |
α4 | Fibronectin, vascular cell adhesion molecule (VCAM) | |
α5 | Fibronectin | |
α6 | Laminins | |
α7 | Laminins | |
α8 | Fibronectin, tenascin | |
α9 | Tenascin | |
α10 | Collagens | |
α11 | Collagens | |
αv | Fibronectin, vitronectin | |
β2 | αL | Intracellular adhesion molecules (ICAMs) |
αM | Fibrinogen, ICAMs | |
αX | Fibrinogen | |
αD | VCAM, ICAMs | |
β3 | αlib | Collagens, fibronectin, vitronectin, fibrinogen, thrombospondin |
αv | Fibronectin, vitronectin, fibrinogen, thrombospondin, osteopontin, tenascin | |
β4 | α6 | Laminins |
β5 | αv | Vitronectin |
β6 | αv | Fibronectin, tenascin |
β7 | α4 | Fibronectin, VCAM, Mucosal addressin cell adhesion molecule (MAdCAM) |
αE | E-cadherin | |
β8 | αv | Collagens, laminins, fibronectin |
Integrins bind specifically to ECM proteins binding sites, such as the RGD sequence that is present in most of them (e.g. fibronectin). The fact that many integrins recognize this sequence is probably the explanation for their overlapping specificity [27, 29]. Besides, integrins can interact with components of the cytoskeleton and signaling molecules through their intracellular domain. Indeed, after binding to the ligand, the integrins also bind to several intracellular anchorage proteins including talin, α-actinin and filamin. Those anchorage proteins can bind directly to actin or to other proteins like vinculin, thereby linking the integrin to actin filaments in the cell cortex. If the proper environmental conditions are in place, this linkage leads to a clustering of integrins and the focal adhesion between the cell and the ECM. The cytoskeletal attachment aids the formation of the integrins cluster providing a stronger aggregate bond [30].
This interaction between integrins and ECM proteins will affect the signal transduction which can induce adhesion, spreading and migration, as well as the expression of transcription factors and specific genes [31]. Besides the recruitment of signaling molecules, integrin activation also leads to changes in the cytoskeleton organization, subsequently affecting cell adhesion and mobility. Signals from different ECM ligands can result in different signaling pathways.
Integrins are promising targets for manipulating cellular and host responses to biomaterials. For instance, controlled integrin binding at the biomaterial interface, in terms of specific integrin receptors may activate specific signaling pathways and adhesive activities that elicit desired cellular and host responses [32]. Therefore, regarding the interaction with a biomaterial, adhesive extracellular ligands for integrins can be adsorbed in the surface from the surrounding environment (e.g. protein adsorption from blood, plasma or serum), deposited by cells on the biomaterial (e.g. fibronectin and collagen deposition), or engineered at the interface [29, 31].
Protein-Biomaterial Interactions
After a biomaterial is implanted in the body, it takes seconds to minutes for proteins to adsorb and cover its surface, forming the so-called conditioning film [11]. Therefore, instead of the original surface of the implanted material, the cells will recognize and interact with this protein layer. It is fair to assume that the adhesion proteins are responsible for converting the biomaterials into biologically recognizable materials. The adsorption of these adhesion proteins is the basis for all the reactions that may further occur in the body [33].
The surface properties of the biomaterials will determine the type, amount and conformation of the adsorbed proteins [2]. The composition of this protein layer can be different, depending on the fluid composition and adsorption time [25]. Besides the composition of the protein layer, the conformation and the orientation of the protein can also change with time [8]. This conditioning protein layer will increase the cell adhesiveness, since the cells have receptors in their membranes that specifically bind to the adhesion proteins. Moreover, the protein layer also increases the cell spreading at the biomaterial surface [6, 10].
Cell-Biomaterial Interactions
Nowadays it is generally accepted that there are no inert biomaterials. Any foreign material that is implanted in the body triggers tissue responses during the healing process that will naturally depend on the nature of the biomaterial and the implant site [3, 34]. Indeed, the host-biomaterial interaction, that controls the biological performance of the implanted device, is a very complex process. Upon device implantation in the body, a wound is formed and the healing process is initiated. The way the implant is more or less accepted by the host, and how well it heals, depends largely on the wound healing process. A cascade of events, common to the body’s reaction mechanism to an implanted material is summarized in Fig. 4.3a.
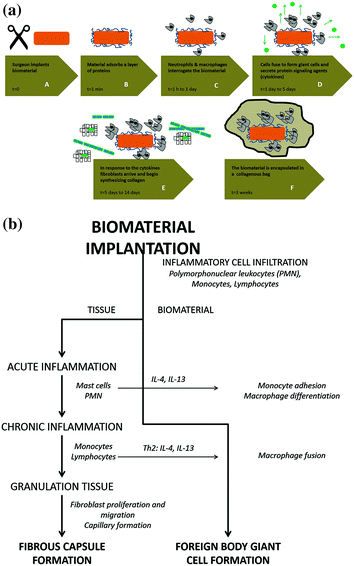
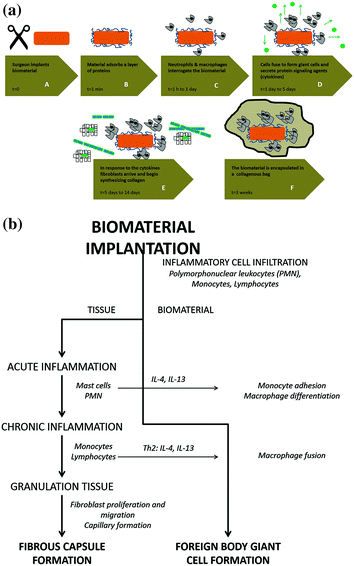
Fig. 4.3
a Host reaction to an implanted biomaterial. (A) The biomaterial is implanted in the body. (B) A layer of serum proteins quickly adsorbs to the implant surface. (C) The neutrophils and macrophages approach and attack the biomaterial that is too large to be ingested. (D) Macrophages cannot digest the implant and fuse into giant cells to engulf it. The giant cells send out cytokines to attract other cells. (E) The fibroblasts arrive and start synthesizing collagen. (F) The implant is entrapped in a collagen bag. (Adapted from Castner and Ratner [8]). b Schematic summary of the sequence of events involved in inflammatory and wound healing responses leading to Foreign Body Giant cell formation. (Adapted from Anderson et al. [19])
Blood-biomaterial interactions, provisional matrix formation, acute and chronic inflammation, granulation tissue development, foreign body reaction, and fibrosis/fibrous capsule development comprise the series of host reactions occurring after device implantation (Fig. 4.3b) [3].
Inflammation
Inflammation is the first response of any vascularized tissue to tissue damage (surgery trauma or presence of a foreign body). Injury and vascular damage triggers the two branches of the blood coagulation system. The first involves activation of platelets, which stick to exposed surfaces or foreign objects and form a plug. The other involves a series of proteolytic reactions, starting with the activation of proteins circulating in the blood by the exposure to a foreign object. This sequence results in the final pathway of conversion of fibrinogen to fibrin and the formation of a clot [8].
Several cellular and non-cellular responses will occur in the first 24 h after the implantation of a device. The first event corresponds to a non-cellular response; it consists in the vasodilatation of the local vessels, which culminates with the increased permeability of the vascular endothelium and the edema formation. RBCs and fluid are present at this stage and can continue to appear with a maximum response in about 3–5 days after injury, which is characteristic of an acute inflammation). If the source of the injury is removed, then the response stops, and the tissue returns to a normal appearance. If the source of injury is not removed and the response continues, then inflammation progresses through various stages to chronic inflammation [4, 19].
Afterwards, a cellular response is observed corresponding to the recruitment of inflammatory cells through molecular signals that act as chemoattractants at the implant site. The first cells migrating to the injury site are the neutrophils, which are responsible for the phagocytosis, engulfing and degradation of the foreign body. Once the neutrophils initiate their function, the monocytes circulating in the blood enter the tissue and differentiate into macrophages, which are also responsible for the phagocytosis and the release of several biochemical factors that can activate other cells. The activated macrophages adhere to the biomaterial and spread on its surface, trying to phagocyte it. When they cannot do the job, they coalesce into very large, multinucleated giant cells, so called foreign body giant cells. This represents chronic inflammation and is usually associated with a large mass of tissue debris that can be harmful to the tissue. In some circumstances, the response is organized into a mass with a characteristic cellular response, including multinucleated giant cells, and is called a granuloma. These can be very painful, harmful, and a cause for concern [3].
It is important to keep these fundamental biological events in mind when evaluating materials for use in or on the body [35, 36]. Acute inflammation is a necessary response for tissue repair; however, a chronic response is harmful. If a material is not tolerated by the host or is toxic, then chronic inflammation will occur. Any biomaterial implanted into the body must be sterile; otherwise, an infection will occur. Sterilization methods may destroy or alter the material’s properties. This is a major issue in developing or adapting materials for use in the biological environment. Infection in the presence of an implanted material is of special concern because often to cure the infection it is necessary to remove the implant [1, 19, 37].
Wound Healing
Whenever there is an injury with destruction of tissue, there must be repair. The first step in the repair process is acute inflammation. Changes occur in the vascular system, and the polymorphonuclear leukocytes (PMN) go into the tissue to clean up the damaged tissue and the foreign material. The platelets get activated and form clots to prevent further leakage of blood into the site. The remaining debris is then phagocytized by the leukocytes. Finally, actual tissue repair begins. In order for the tissue to function, there must be a blood supply to the small vessels starting to grow into the wound. Tissue cells, called fibroblasts, come in and start to synthesize collagen. This forms a structural network called granulation tissue [38].
Healing in the Presence of a Biomaterial
Upon insertion of a biomaterial into the body, all the features above described are involved. First, there is damage to the tissue during the insertion, and then an acute inflammatory response occurs. This is followed by the wound healing response that can proceed normally if the biomaterial is there for a short period of time, otherwise the response is distinct. The normal tissue repair cannot take place since there is a foreign body in the way. The first reaction of the body is to try to eliminate it. At this stage, the phagocytes come in and the acute inflammatory response may progress to chronic response. If it is not a degradable material or prone to phagocytosis, then the reaction will follow one of the two paths. The usual response consists in the formation of a fibrous capsule around the biomaterial by the fibroblasts in an attempt to eliminate it from the body. It is generally accepted that the thickness of the fibrous capsule is an indication of the material’s biocompatibility, i.e. a thinner capsule corresponds to a more biocompatible material [38]. There are two types of factors that can influence the wound healing process, the intrinsic factors and the extrinsic factors. The bulk nature of the biomaterial, its porosity, roughness and changes in the surface chemistry are intrinsic factors of the implant [39]. The extrinsic factors are, for example, the surgical procedure, the condition of the patient (diabetic, immunocompromised) and the anatomical location of the implant [4]. The less common response is for the inflammatory process to continue as a chronic inflammatory response and to progress to giant cells and granulomas. This occurs when the material is not biocompatible, and the host reaction is still trying to neutralize it.
At this stage some problems can occur associated with the host reaction to the biomaterial ultimately leading to infection. The formation of the fibrous capsule is an indication that the material is biocompatible and will occur as an early step in healing. Fibrous capsule formation needs to be considered when predicting device function [19]. For instance, if the device is to serve as a drug delivery system, the formation of the fibrous capsule may alter the permeability of the device and the diffusion of the drug so that the function of the device will not be anticipated from laboratory studies. Formation of a fibrous capsule generally does not occur with porous or textured materials [15]. If the interstices are large enough, local vascularized tissue will grow into the pores rather than form the capsule. Long-term percutaneous catheters have been coated with velour to facilitate ingrowth and anchorage. Vascular prostheses made of fabric demonstrate ingrowth of vascular-like tissue [37]. Joint replacement prostheses are sometimes coated with metal beads or wire mesh to facilitate ingrowth of bone and biological anchorage [37]. It is important to notice that acute inflammation and wound healing are necessary steps in the implantation of biomaterials and devices that enter the body, hence the effect of these responses on the function of the device must be considered.
Infection
Whenever there is damage to the skin or mucous membranes and bacteria can enter, there is the risk of infection [40]. The occurrence of infection will prevent the resolution of inflammation, and a chronic inflammatory response will arise. If there is a chronic inflammatory response, the wound healing response will not be completed. The combination of the injury and the presence of the foreign material will initiate the inflammatory response. It is well known that the presence of a foreign body greatly increases the infection risk and markedly decreases the number of bacteria required to cause an infection from 106 to 102. Not only is there an increased risk of infection but also the infection will be difficult to cure, most often only through the removal of the device [3]. The consequences of this depend on the need for the device. Removal of sutures may cause little impairment to healing, whereas removal of the total artificial heart would result in death [15].
Immune Response
The immune system is an important and complex protective system that reacts specifically and with memory [42]. As previously mentioned, the lymphocytes are the key element in the immune system. Although the immune system is an important defence mechanism, sometimes it can cause harm to the host. These reactions are called allergy or autoimmunity [19].
Allergic responses occur to foreign substances through a variety of mechanisms. One type of allergy is called atopic or type I. An inflammatory response occurs, and the nature of the reaction depends on the site. This type of reaction must be avoided by minimizing use of materials with substances that stimulate the reaction [3]. The current concern in this area is with latex materials, such as surgical gloves, which contain a protein that may cause this type of allergy [4]. A second type of reaction is called contact dermatitis (type IV). It is important in evaluating biomaterials to determine that stimulation of allergic responses similar to this does not occur, and it is generally the degradation products from the materials that are of concern [1]. Care is taken to avoid the use of chemicals that are known to cause allergy or to minimize their release into the body. Some of the metals, such as nickel and chromium, are common causes of allergy when contacted as metal salts [1]. Allergic reactions to metallic devices are a concern, but unless there is corrosion and release of metal ions, there will not be allergic responses.
The issue of autoimmunity is also important in the selection of biomaterials and evaluation of responses. In autoimmune reactions, there is an immune reaction that causes damage to the host tissue. This occurs because the material altered the host tissue in some manner or the immune response to the biomaterial also reacted with the host tissue [19, 42].
Biocompatibility Determined by Cell-Material Interactions
Currently, a biocompatible material is defined as a material that is able to perform an appropriate host response in a specific application [1]. Nevertheless, for many years the term “biocompatibility” was associated to the biological “inertness” of the material. The main goal behind the design of such biological inert materials was to reduce or virtually eliminate any unfavorable immune response to the foreign body or biomaterial. However, for some applications it is required that cells interact with such biomaterials, for example to promote cell adhesion and proliferation [4], and to promote cells and tissue ingrowth. In these cases, the strategy adopted to improve the material’s biocompatibility is to incorporate specific bioactive molecules on the polymer surfaces in order to promote or support of a favorable cell-material interaction [5, 10]. Therefore, for searching biomaterials able to provide the best performance in each application it is crucial to understand the cell-material interactions, namely the chemical, biochemical, physiological, physical or other mechanisms involved in such interactions, as well as their consequences [2, 37, 41].
Effect of the Materials Properties on Cell Behaviour
Today, there are already several (bio)materials available for use in tissue engineering applications and as medical implants [43–45], these include dental fillings, coating for tablets or capsules, contact lenses, kidney dialyzers, vascular grafts, cardiac pacemakers, maxillofacial prosthesis, to name a few examples. A wide variety of biodegradable polymers (natural or synthetic), bioactive ceramics, wear-resistant metal alloys have become available for use in a variety of human conditions [46–50]. From the previous sections it is reasonable to assume that all materials to be used as biomaterials, medical devices, or prostheses inevitably undergo a host tissue response, following implantation. This occurs as a consequence of the interaction of cells and tissues with the outermost surface layers of the materials. One of the first phenomena observed in tissue culture assays was the response of cells to the topography of the substratum to which they were attached [51, 52], these surfaces exhibited a topography measured on the micro scale. Subsequent research has shown that, along with topography, the surface chemistry, microstructure, porosity, surface area, thermal, mechanical and electrical properties of the biomaterial play a significant role on cell behaviour [43, 44, 47, 50, 53–60].
Morphological Properties
Surface Topography
Surface roughness (Fig. 4.4a) can essentially be divided into nano-roughness (<100 nm), micro-roughness (100 nm–100 μm) and macro-roughness (from 100 μm to several millimetres) [61]. The cells response to roughness will vary with the cell type and roughness scale (i.e. size, shape and distribution on the materials’ surface). Hence, surface roughness regulates the biological response of cells and tissues that are in contact with the implanted material. It provides cues to promote cell adhesion, orientation, migration and production of ECM. For instance, cells grown on micro-rough surfaces spread more and differentiate better than on smooth ones, as shown by their gene expression [62–64]. Also, on grooved materials, cells can orient themselves in the direction of grooves and proliferate faster than on a smooth surface [43, 65–70].
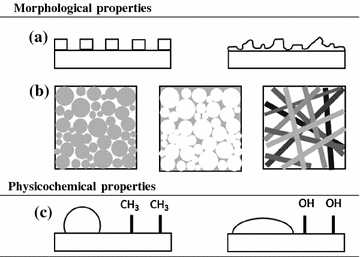
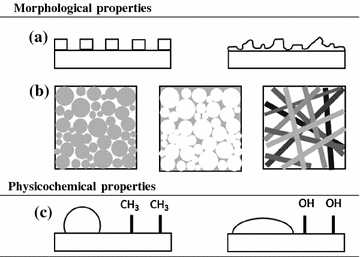
Fig. 4.4
Relevant properties of biomaterials. Morphological properties: a surface topography (etched surfaces can be obtained with either specific patterns (left) or irregular (right); b porosity (the white regions inside the squares represent the porous (void) fraction of the scaffolds); c surface charge and hydrophobicity: a water droplet on a hydrophobic surface (Table 4.4) will have a higher contact angle (left) than on a hydrophilic surface (right)
Nanotopography may provide superior biomimetic cell-modulating cues, as it may further resemble the natural ECM environment in which cells reside and interact. It has been shown that nanopatterned surfaces can improve protein adsorption and cellular response, since as on rougher surfaces, the focal adhesion points are located at cell edges, where the contact with the materials’ surface takes place [61, 71–77].
Porosity
Porous structures (Fig. 4.4b) typically consist of irregularly shaped voids with interconnecting channels (voids). The pore size, porosity (the fraction of void volume) and surface area (also presented as a surface-to-volume ratio parameter when characterizing a biomaterial), pore shape, wall morphology (tortuosity) and pore interconnectivity are important requisites for a scaffold in tissue engineering applications. The tailoring of these properties allows for rapid cell attachment and proliferation, improve nutrient and cell transfer to the scaffolds’ center (cell and tissue ingrowth), as well as metabolite dispersal and new tissue formation [78–84]. The open pore structure and interconnected porosity have also been found to disrupt fibrous tissue deposition, minimize the formation of foreign-body capsules, improve tissue healing, and increase vascularization of the tissue surrounding the implant [85–90]. Pore size (microporosity: pore size <10 µm; macroporosity: pore size >50 µm) can be tailored to meet specific applications (Table 4.3); for instance the optimum size for neovascularization is around 5 µm; whereas for fibroblast ingrowth is in the range of 5–15 µm; for hepatocytes ingrowth close to 20 µm; for the regeneration of adult mammalian skin around 20–125 µm; for osteoid ingrowth around 40–100 µm and for bone regeneration around 100–350 µm. On the other hand, fibrovascular tissues require pores sizes greater than 500 µm for a rapid vascularization and for the survival of the transplanted cells [91, 92].
Table 4.3
Optimal pore size for cell infiltration and host tissue ingrowth (adapted from Chang and Wang [93])
Cell/tissue type | Optimal pore size (μm) | Scaffold materiala | Reference |
---|---|---|---|
Human skin fibroblasts | <160 | PLLA/PLGAa | Yang et al. [94] |
Bone | 450 | PMMAa | Ashman and Moss [95] |
Fibrocartilaginous tissue | 150–300 | Polyurethane | de Groot et al. [96] |
Adult mammalian skin cells | 20–125 | Collagen-glycocaminoglycan | Yannas et al. [97] |
Osteogenic cells | 100–150 | Collagen-GAGa | O’Brien et al. [56] |
Smooth muscle cells | 60–150 | PLLA | Zeltinger et al. [98] |
Endothelial cells | <80 | Silicon nitride | Salem et al. [99] |
Physico-Chemical Properties
Surface Hydrophobicity
One of the most common techniques used to measure the surface energy of a material is the determination of contact angles, whereby the angle made by a drop of liquid deposited on a surface correlates with its hydrophobicity (Fig. 4.4c). The size and shape of the liquid droplet can be explained as a balance between the force with which the molecules of the liquid drop are being attracted to each other (designated by cohesive force) and, on the other hand, the attraction of the liquid molecules for the surface (adhesive force). The balance between these two opposing forces is expressed by the contact angle value (θ), which in turn translates the hydrophobicity of a given surface. Consequently, relatively more water wettable surfaces (i.e. exhibiting lower θ) are termed hydrophilic (high surface energy); conversely less wettable surfaces (with higher θ) are termed hydrophobic (low surface energy). To establish a borderline between these two terms, it is generally agreed that the criterion of a θ = 65° separates between the two regimes [100–105].
In the previous sections it was already explained that many proteins adsorb onto an implant surface immediately upon contact with it. This event modulates subsequent cell adhesion and/or foreign-host response (protein adsorption is the first step in the integration of an implanted device with its surrounding tissues). The dominant response will depend largely on the hydrophobicity (also referred to as surface energy or wettability) of the scaffold. The materials hydrophobicity thus affects protein adsorption, platelet adhesion/activation, blood coagulation and cell and bacterial adhesion. For instance, the adsorption of serum proteins (fibrinogen, fibronectin or vitronectin) later affect the adhesion of leukocytes, macrophages or platelets by preventing ECM proteins adsorption, ultimately leading to fibrous encapsulation; thus delaying or even preventing proper cell adhesion. Also, surface wettability (hydrophobicity) was found to regulate cytoskeletal organization and cell morphology. As a general rule, the more hydrophilic a surface is, the more cells adhere to it. Conversely, hydrophobic surfaces are more prone to protein-adsorption. This occurs due to the strong hydrophobic interactions between the material’s surface and the protein’s hydrophobic groups [6, 93, 106–113]. With respect to contact angle values, literature shows that polymer surfaces exhibiting moderate wettability (i.e. θ in the range 40–70°) enhance cell adhesion [106, 114, 115].
In the biomedical field, protein-surface interactions play key roles for the assembly of interfacial protein constructs, such as biosensors, activators and other functional components at the biological/electronic interface. In analytical sciences, non-specific protein adsorption on sensor surfaces, protein chips, or assay platforms represents a serious problem as adsorbed proteins are responsible for the degradation and thus decrease of the device performance. For example, in biosensors for in situ monitoring of cell culture or blood glucose levels in diabetic patients, specificity and sensitivity levels, and durability depend greatly on the protein adsorption onto the sensor surface. Likewise, in polymeric drug matrix delivery systems the accumulation of adsorbed proteins may impair the diffusion of the drug. In the design of biocompatible materials for surgical implants, adsorption of fibrinogen in particular should be suppressed, to avoid blood agglutination [116–118].
Surface Charge
Surface chemistry is very important for the physiological interactions with biomaterials and it is closely related to surface energy (hydrophobicity); the surface free energy of a material originates from its surface functional groups and electrical charges. The material’s surface chemistry mediates cell response given that surface chemistry directly impacts on protein adsorption and interaction (i.e. surface chemistry affects the amount and conformation of the adsorbed proteins). All these factors play crucial roles in the later cell interactions and functions [119–122]. Table 4.4 lists some of the material’s most common surface groups and describes how they affect proteins, cells and tissue interactions.
Table 4.4
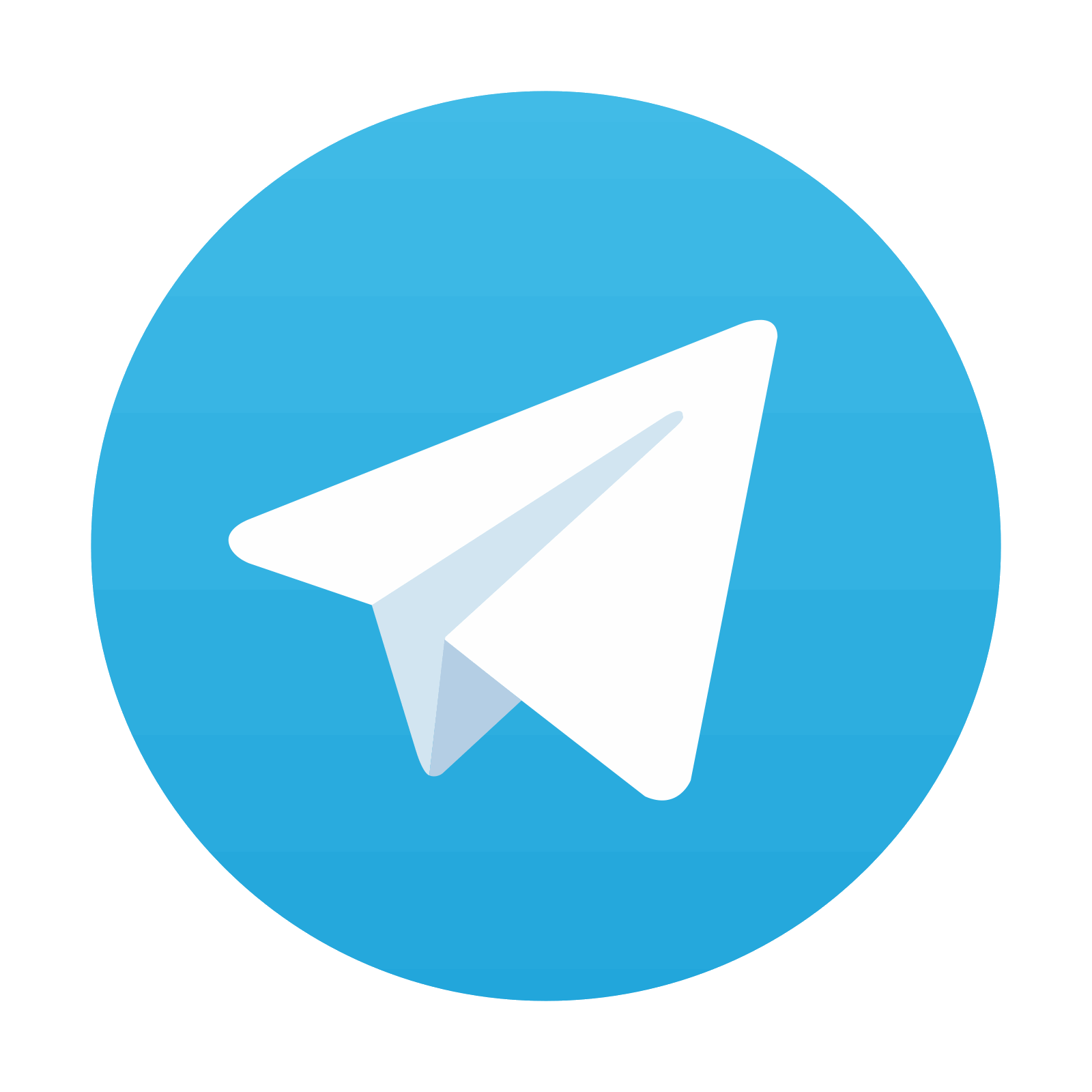
The effect of material surface functional groups on proteins, cells and tissue interactions (adapted from Barbosa et al. [127], Faucheux et al. [128], Kamath et al. [129] Keselowsky et al. [120, 130], Kidoaki and Matsuda [131], Lan et al. [132], Lorenz et al. [133], Schmidt et al. [122], Tang et al. [134], Thevenot et al. [2], Verena et al. [135])
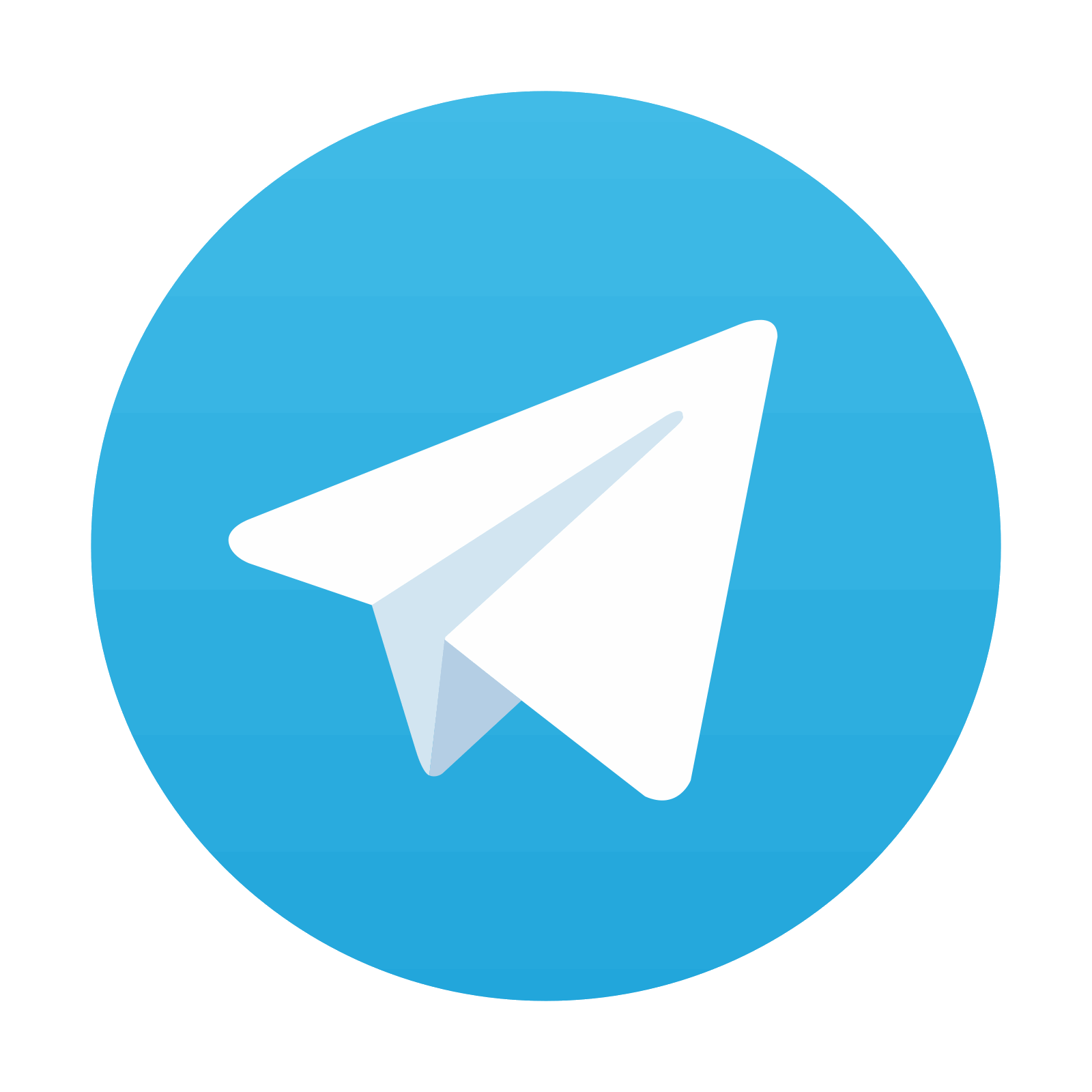
Stay updated, free articles. Join our Telegram channel
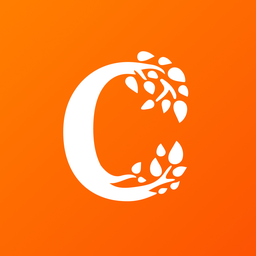
Full access? Get Clinical Tree
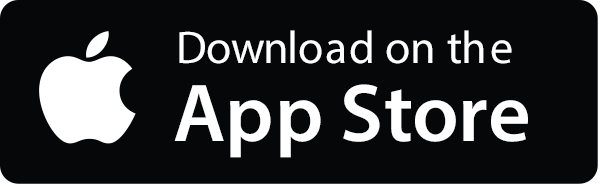
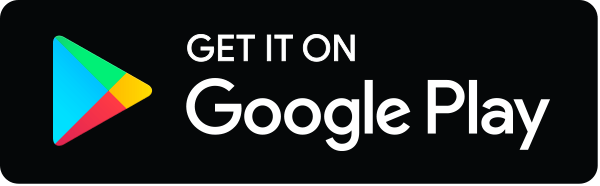
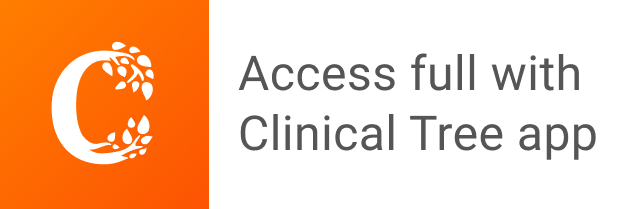