Chapter 5 Disorders of growth, differentiation and morphogenesis
DEFINITIONS
Growth
Types of growth in a tissue (Fig. 5.1A) are:
Differentiation
Differentiation is the process whereby a cell develops an overt specialised function or morphology that distinguishes it from its parent cell. There are many different cell types in the human body, but all somatic cells in an individual have identical genomes. Differentiation is the process by which genes are expressed selectively and gene products act to produce a cell with a specialised function (Fig. 5.1B). After fertilisation of the human ovum, and up to the eight-cell stage of development, all of the embryonic cells are apparently identical. Thereafter, cells undergo several stages of differentiation in their passage to fully differentiated cells, such as, for example, the ciliated epithelial cells lining the respiratory passages of the nose and trachea. Although the changes at each stage of differentiation may be minor, differentiation can be said to have occurred only if there has been overt change in cell morphology (e.g. development of a skin epithelial cell from an ectodermal cell), or an alteration in the specialised function of a cell (e.g. the synthesis of a hormone).
NORMAL AND ABNORMAL GROWTH IN SINGLE TISSUES
Within an individual organ or tissue, increased or decreased growth takes place in a range of physiological and pathological circumstances as part of the adaptive response of cells to changing requirements for growth. In both fetal and adult life, tissue growth depends upon the balance between the increase in cell numbers, due to cell proliferation, and the decrease in cell numbers, due to cell death. Non-proliferative cells are termed ‘quiescent’; such cells differentiate and adopt specific phenotypes capable of carrying out their specific function (Fig. 5.2).
Regeneration
The high regenerative potential of the skin is exploited in the treatment of patients with skin loss due to severe burns. The surgeon removes a layer of skin which includes the dividing basal cells from an unburned donor site, and fixes it firmly to the burned graft site where the epithelium has been lost (Ch. 6). Dividing basal cells in the graft and the donor site ensure regeneration of squamous epithelium at both sites, enabling rapid healing in a large burned area where regeneration of new epithelium from the edge of the burn would otherwise be prolonged.
Permanent cells normally divide only during fetal life, but their active stem cells do not persist long into post-natal life, and they cannot be replaced when lost. Cells in this category include neurones, retinal photoreceptors and neurones in the eye, cardiac muscle cells and skeletal muscle (although skeletal muscle cells do have a very limited capacity for regeneration).
The cell cycle
Successive phases of progression of a cell through its cycle of replication are defined with reference to DNA synthesis and cellular division. Unlike the synthesis of most cellular constituents, which occurs throughout the interphase period between cell divisions, DNA synthesis occurs only during a limited period of the interphase; this is the S phase of the cell cycle. A further distinct phase of the cycle is the cell-division stage or M phase (Fig. 5.3) comprising nuclear division (mitosis) and cytoplasmic division (cytokinesis). Following the M phase, the cell enters the first gap (G1) phase and, via the S phase, the second gap (G2) phase before entering the M phase again. Although initially regarded as periods of inactivity, it is now recognised that these ‘gap’ phases represent periods when critical processes occur, preparing the cells for DNA synthesis and mitosis.
Molecular events in the cell cycle
Cell division is a highly complex process and cells possess elaborate molecular machinery to ensure its successful completion. A number of internal ‘checkpoints’ exist to ensure that one phase is complete before the next commences (Fig. 5.3). This is vital to ensure, for example, that DNA replication has been performed accurately and that cells do not divide before DNA replication is complete. The various proteins and enzymes that carry out DNA replication, mitotic spindle formation, etc. are typically only present and active during the appropriate phases of the cycle. The timely production and activation of these proteins is regulated by the activity of a family of evolutionarily conserved proteins called cyclin dependent kinases (CDKs), which activate their target proteins by phosphorylation. The activity of CDKs is, in turn, regulated by a second family of proteins, the cyclins. Transitions from one phase of the cycle to the next are initiated by rises in the levels of specific cyclins. The transition from G0 to G1 at the initiation of the cell cycle, for example, is triggered by external signals such as growth factors leading to rises in the levels of cyclin D. Problems during cell division, such as faulty DNA replication, result in rises in the levels of a third family of proteins, the CDK inhibitors (CDKIs), which can prevent CDKs from triggering the next phase of cell division until the issue is resolved. In the face of major failures, cells will typically initiate apoptosis rather than permit the generation of improperly formed progeny. Damage to the genes that encode proteins involved in the regulation of cell-cycle progression is seen in many cancers (Ch. 11).
Therapeutic interruption of the cell cycle
Many of the drugs used in the treatment of cancer affect particular stages within the cell cycle (Fig. 5.4). These drugs inhibit the rapid division of cancer cells, although there is often inhibition of other rapidly dividing cells, such as the cells of the bone marrow and lymphoid tissues. Thus, anaemia, a bleeding tendency and suppression of immunity may be clinically important side-effects of cancer chemotherapy.
Cell death in growth and morphogenesis
It seems illogical to think of cell death as a component of normal growth and morphogenesis, although we recognise that the loss of a tadpole’s tail, which is mediated by the genetically programmed death of specific cells, is part of the metamorphosis of a frog. It is now clear that such cell death has an important role in human development and in the regulation of tissue size throughout life. Alterations in the rate at which cell death occurs are important in situations such as hormonal growth regulation, immunity and neoplasia.
Apoptosis
Morphogenetic apoptosis is involved in alteration of tissue form. Examples include:
Regulation of apoptosis
Apoptosis may be triggered by external signals, such as detachment from the extracellular matrix, the withdrawal of growth factors, or specific signals from other cells. This mode of activation of apoptosis is called the extrinsic pathway. By contrast, the intrinsic pathway is activated by intracellular signals, such as DNA damage or failure to conduct cell division correctly. Although apoptosis can be induced by diverse signals in a variety of cell types, a few genes appear to regulate a final common pathway. The most important of these are the members of the bcl-2 family (bcl-2 was originally identified at the t(14;18) chromosomal breakpoint in follicular B-cell lymphoma, and it can inhibit many factors that induce apoptosis). The bax protein (also in the bcl-2 family) forms bax–bax dimers which enhance apoptotic stimuli. The ratio of bcl-2 to bax determines the cell’s susceptibility to apoptotic stimuli, and constitutes a ‘molecular switch’ which determines whether a cell will survive, leading to tissue expansion, or undergo apoptosis.
Increased growth: hypertrophy and hyperplasia

The response of an individual cell to increased functional demand is to increase tissue or organ size (Fig. 5.6) by:
An important component of hyperplasia, which is often overlooked, is a decrease in cell loss by apoptosis; the mechanisms of control of this decreased apoptosis are unclear, although they are related to the factors causing increased cell production (Fig. 5.7).
Physiological hypertrophy and hyperplasia
Examples of physiologically increased growth of tissues include:
Pathological hypertrophy and hyperplasia
Many pathological conditions are characterised by hypertrophy or hyperplasia of cells. In some instances, this is the principal feature of the condition from which the disease is named. The more common examples are summarised in Table 5.1. For more detail, consult the relevant chapters.
Table 5.1 Examples of non-regenerative hypertrophy and hyperplasia
Organ/tissue | Condition | Comment |
---|---|---|
Myocardium | Right ventricular hypertrophy | Response to pulmonary valve stenosis, pulmonary hypertension or ventricular septal defect (Ch. 13) |
Left ventricular hypertrophy | Response to aortic valve stenosis or systemic hypertension (Ch. 13) | |
Arterial smooth muscle | Hypertrophy of arterial walls | Occurs in hypertension (Ch. 13) |
Capillary vessels | Proliferative retinopathy | Complication of diabetes mellitus (Ch. 26) |
Bone marrow | Erythrocyte precursor hyperplasia | Response to increased erythropoietin production (e.g. due to hypoxia) (Ch. 23) |
Cytotoxic T-lymphocytes | Hyperplastic expansion of T-cell populations | Involved in cell-mediated immune responses (Ch. 9) |
Breast | Juvenile hypertrophy (females) | Exaggerated pubertal enlargement (Ch. 18) |
Due to high oestrogen levels (e.g. in cirrhosis, iatrogenic, endocrine tumours) (Ch. 18) | ||
Gynaecomastia (males) | ||
Prostate | Epithelial and connective tissue hyperplasia | Relative excess of oestrogens stimulates oestrogen-sensitive central zone (Ch. 20) |
Thyroid | Follicular epithelial hyperplasia | Most commonly due to a thyroid-stimulating antibody (Graves’ disease) (Ch. 17) |
Adrenal cortex | Cortical hyperplasia | Response to increased ACTH production (e.g. from a pituitary tumour or, inappropriately, from a lung carcinoma) (Ch. 17) |
Myointimal cells | Myointimal cell hyperplasia in atheromatous plaques | Myointimal cells in plaques proliferate in response to platelet-derived growth factor (Ch. 13) |
Hyperplasia in tissue repair
Angiogenesis is the process whereby new blood vessels grow into damaged, ischaemic or necrotic tissues in order to supply oxygen and nutrients for cells involved in regeneration and repair (the term ‘vasculogenesis’ should be reserved specifically for the blood vessel proliferation that occurs in the developing embryo and fetus). In response to local tissue damage, vascular endothelial cells within pre-existing capillaries are activated by angiogenic growth factors such as vascular endothelial growth factor (VEGF), released by hypoxic cells or macrophages. These activated endothelial cells then migrate towards the angiogenic stimulus to form a ‘sprout’. Cell migration is facilitated by the secretion of enzymes including the matrix metalloproteinases, which selectively degrade extracellular matrix proteins. Adjacent sprouts connect to form vascular loops, which canalise and establish a blood flow. Later, mesenchymal cells—including pericytes and smooth muscle cells—are recruited to stabilise the vascular architecture, and the extracellular matrix is remodelled.
Skin
The healing of a skin wound is a complex process involving the removal of necrotic debris from the wound and repair of the defect by hyperplasia of capillaries, myofibroblasts and epithelial cells. Figure 5.8 illustrates some of the key events, most of which are mediated by growth factors.
Liver
In severe chronic hepatitis (Ch. 16) extensive hepatocyte loss is followed by scarring, as is the case in the skin or other damaged tissues. Like epidermal cells in the skin, hepatocytes have massive regenerative potential and surviving hepatocytes may proliferate to form nodules. Hyperplasia of hepatocytes and fibroblasts is presumably mediated by a combination of hormones and growth factors, although the mechanisms are far from clear. Regenerative nodules of hepatocytes and scar tissue are the components of cirrhosis of the liver.
Heart
Myocardial cells are permanent cells (i.e. they remain permanently in G0 and cannot enter G1), and so cannot divide in a regenerative response to tissue injury. In myocardial infarction, a segment of muscle dies and, if the patient survives, it is replaced by scar tissue. As the remainder of the myocardium must work harder for a given cardiac output, it undergoes compensatory hypertrophy (without cell division) (Fig. 5.9). Occasionally, there may be right ventricular hypertrophy as a result of left ventricular failure and consequent pulmonary hypertension.
Decreased growth: atrophy
Atrophy occurs in both physiological and pathological conditions.
Physiological atrophy and involution
Physiological atrophy occurs at times from very early embryological life, as part of the process of morphogenesis. The process of atrophy (mediated by apoptosis of cells) contributes to the physiological involution of organs such as the thymus gland in early adult life, and late old age is accompanied by atrophy of various tissues (Table 5.2).
Table 5.2 Tissues involved in physiological atrophy and involution
Embryo and fetus | Early adult |
Branchial clefts | Thymus |
Notochord | |
Thyroglossal duct | Late adult and old age |
Müllerian duct (males) | Uterus, endometrium (females) |
Wolffian duct (females) | Testes (males) |
Bone (particularly females) | |
Neonate | Gums |
Umbilical vessels | Mandible (particularly edentulous) |
Ductus arteriosus | Cerebrum |
Fetal layer adrenal cortex | Lymphoid tissue |
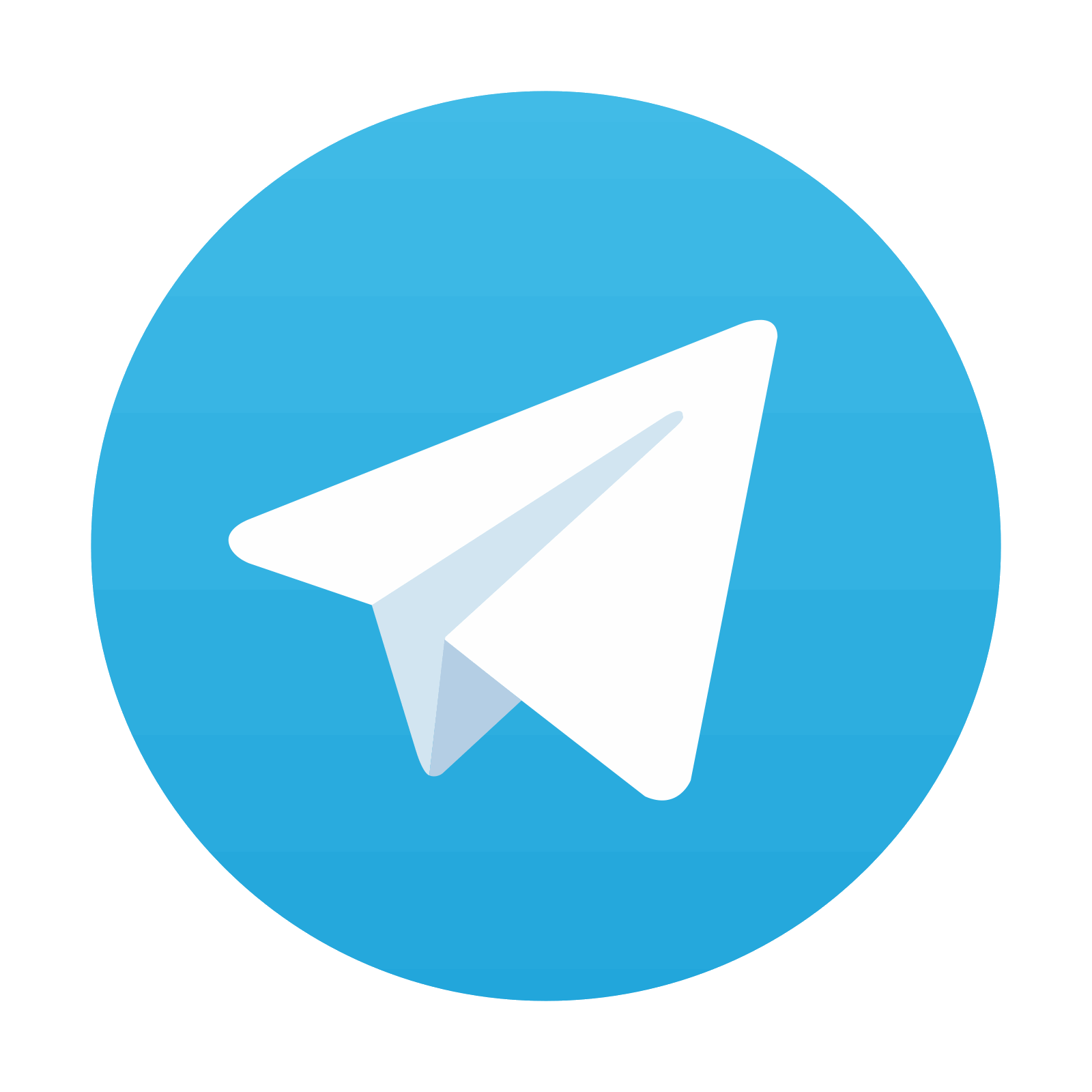
Stay updated, free articles. Join our Telegram channel
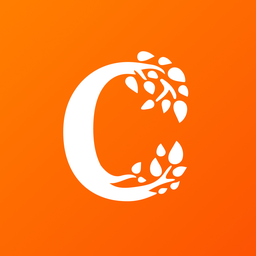
Full access? Get Clinical Tree
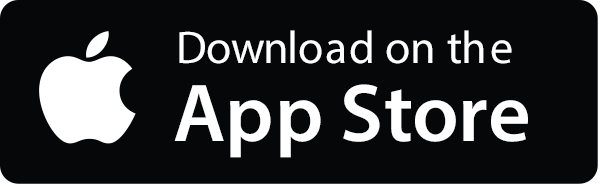
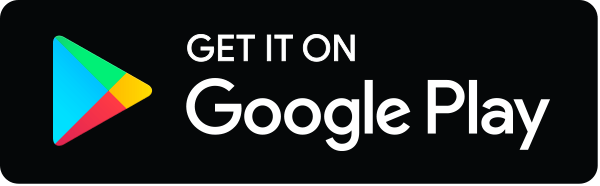