4.1 Introduction
Manufacturing of suspension-type metered-dose inhaler (MDI) and dry powder inhaler (DPI) products for pulmonary drug administration requires powders with desirable characteristics, including particle size, shape, density, crystallinity, surface morphology, and surface energy. These characteristics will affect the physical stability of the aerosol formulations and the interactions between different particles and between particles and the inhaler during aerosolization. Drug powders for inhalation have traditionally been produced by crystallization followed by milling to reduce the particles to the size range required for aerosol formulation and delivery to the lungs. While such a production approach to inhalation products may have been sufficient in the past, it is not suitable for producing powders with the required flow and dispersion characteristics to meet the need for enhanced powder performance. Various methods that have been explored or are in an advanced stage of development are examined in this chapter.
4.2 Conventional Crystallization and Milling
Crystallization involves several main steps: supersaturation, nucleation, and crystal growth. Both nucleation and crystal growth depend on the level of supersaturation. Industrial crystallization usually takes place within chemical reactors, which can be scaled up for large-batch production. Since it is not easy to achieve a uniform supersaturation level inside the reactor, conventional crystallization suffers from disparity in the rate of nucleation and the subsequent crystal growth. This will lead to poor control over the particle shape and size distribution. In general, this process tends to produce crystals >10 μm, with a broad size distribution, which will not be suitable for inhalation to the lungs.
After crystallization, the precipitate is collected by filtration and is dried in an oven at a set temperature. To reduce the particle size of the crystals, milling (e.g. fluid-energy/air-jet-milling or ball milling) is commonly employed in the pharmaceutical industry. In ball milling, powder contained inside a drum is grounded by rolling balls of various sizes as the drum rotates. In fluid-energy milling, compressed air is fed along with the powder into the mill, where the air accelerates and causes attrition of the particles by collision. Due to the high energy imparted to the particles in the milling process, they become partially amorphous, with reduced crystallinity (and hence attain a higher energy state than the fully crystalline materials). These particles can also carry electrostatic charge, resulting from friction or contact with the mill surface—a process known as trioboelectrification. Having higher surface energy and charge, these particles are very cohesive, making the powder difficult to handle. Furthermore, the powder will recrystallize from the partially amorphous state under elevated temperature and humidity conditions, causing potential instability problems during manufacturing and storage. An additional problem is that malleable materials such as inhaled steroid drugs (e.g. triamcinolone acetonide) are difficult to mill [1]. Despite these limitations, crystallization and milling are established techniques and have been widely used in the past for inhalation products [2–4].
However, because of the potential limitations of the conventional production process, alternative methods have emerged for the manufacture of powders with enhanced performance, increasing the amount of fine particles in the aerosol—with less device retention—and reducing dose variability.
4.3 Specialized Milling
Micronization may produce partially amorphous materials with a surface charge, causing particle agglomeration. These problems can be dealt with by specialized milling methods.
4.3.1 Fluid-Energy Milling at Elevated Humidity
In order to reduce the amorphous content in the material produced by milling, the milling can be carried out at elevated humidity. The absorbed moisture functions as a plasticizer to lower the glass transition temperature of the amorphous material, hence facilitating in situ crystallization during the milling process. The milled products have been reported to be predominantly crystalline, with particle size distributions similar to those produced by the conventional milling process [5]. The setup involves controlling the relative humidity (e.g. 30–70%) of the milling chamber by humidifying the feed gas (e.g. by superheated steam, to minimize condensation) used to mill the powder [5].
4.3.2 Wet-Milling Nanotechnology
Nanocrystal Technology (Elan Drug Technologies, King of Prussia, PA, USA) is an aqueous-based milling process used to reduce particle size to below 400 nm. A conventional ball mill can be used for the process, and the materials selected for the grinding media (e.g. glass, zirconium oxide) have been reported not to be crucial [6]. However, the grinding media should preferably be ≤1 mm in order to be effective in attrition and to impart less wear to the mill [6]. Milling in a water medium causes amorphous-to-crystalline transformation, and the resulting powder is physically more stable than that produced by dry milling.
Budesonide and other compounds were milled to nanosized particles, which were then spray- or freeze-dried for use in MDI, DPI, or nebulizer systems [7, 8]. A surface modifier (e.g. PVP, lecithin, cellulose derivatives) is usually added during or after milling to prevent agglomeration of the nanoparticles. Although these stabilizers can be of generally-recognized-as-safe (GRAS) materials, long-term inhalation can be a concern unless proven safe. Another major drawback of wet milling is that, depending on the type of mill and the drug, a lengthy processing time may be required (5 days or longer).
Another process used for wet milling is high-pressure (piston-gap) homogenization, which uses cavitation forces and impact or shear forces to reduce particle size. In this technique, the drug powder to be milled is dispersed in an aqueous solution containing a surface modifier (surfactant or polymer) and then stirred at high speed to form a suspension. It is then milled in a piston-gap homogenizer to <5 μm. This method has been used to produce budesonide nanosuspension containing drug particles of 500–600 nm suitable for nebulization [9]. In addition to the requirement for preprocessing the powder into a suspension, low suspension viscosity (hence low solid loading of <10%) may be a limitation of this method.
4.4 Solvent Precipitation
Inhalable particles can potentially be obtained by rapid precipitation from aqueous solutions using antisolvents. A number of processes have emerged to control the nucleation rate and crystal growth, so as to reproducibly generate particle size in the micron range for aerosol delivery.
4.4.1 Sono-Crystallization
Ultrasonic radiation has been applied to control the precipitation process [10]. The setup can simply comprise an ultrasound probe in a mechanically stirred reaction tank, where the antisolvent is mixed with the drug solution to precipitate the fine drug particles. The ultrasound frequency is crucial and 20–25 kHz (or higher) has been reported to be suitable for setups similar to the one shown in Figure 4.1 [10]. The ultrasonic waves help to nucleate and crystallize particles more evenly and faster. Nucleation and crystal-growth processes can be achieved in a short time because ultrasound reduces the width of the metastable zone and so nucleation can start at a lower level of supersaturation.
Figure 4.1 Schematic diagram of the sono-crystallization process. Reproduced with permission from Chan H-K, Kwok PCL. Production methods for nanodrug particles using the bottom-up approach. Advanced Drug Delivery Reviews 2011;63:406–416.
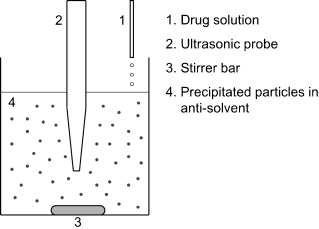
The sonication process is based on cavitation resulting from the constant creation, growth, and implosive disintegration of bubbles in liquid. Apart from the fast procedure, sonication offers further advantages: (1) it produces a smaller crystal size and narrower size distribution of the products than a conventional crystallization process, because crystal growth occurs at a lower supersaturation level, where initial growth is less rapid; (2) it is relatively cheap; (3) it can be carried out at ambient conditions; and (4) the reaction vessel required is relatively small and simply shaped, which makes the cleaning process easy.
The frequency of the ultrasonic wave affects the amount of cavitation (i.e. creation of bubbles) per unit volume per unit time and bubble size. The bubble radius is inversely proportional to the frequency. At a given power, the amount of cavitation increases with increasing frequency, but the bubbles become smaller. Their implosions result in less energy being released, because bubble energy is proportional to the square of the bubble radius. Input power (amplitude) determines the size of the bubbles created. At a constant frequency, higher power (amplitude) will create bigger bubbles. When these bubbles burst, the intensity of the cavitation energy released is higher. At higher frequency, although the cavitation energy intensity is low, the number of cavitation implosions per unit volume per unit time is high. This could possibly lead to the enhancement of localized micromixing and hence to a narrow distribution of smaller particle size, as observed in sodium chloride particles for inhalation [11] (Figure 4.2).
Figure 4.2 Inhalable sodium chloride particles produced by sono-crystallization. Adapted with permission from Tang P, Chan H-K, Tam E, de Gruyter N, Chan J. Preparation of NaCl powder suitable for inhalation. Industrial & Engineering Chemistry Research 2006;45(12):4188–4192. Copyright (2006) American Chemical Society
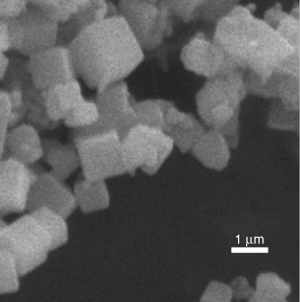
Examples of antiasthmatic drugs prepared using sono-crystallization techniques include fluticasone propionate and salmeterol xinafoate [10]. This method should be applicable to the preparation of other inhalable compounds, such as salbutamol sulfate, beclomethasone dipropionate, budesonide, and formoterol fumarate.
In other applications, ultrasound has been employed either to induce crystallization inside dispersed solution droplets in a liquid medium, or to produce a stable emulsion followed by crystallization in the droplets induced by mass or heat transfer (such as cooling or diffusion of an antisolvent into the droplets) [12].
4.4.2 Microprecipitation by Opposing Liquid Jets and Tangential Liquid Jets
In this method, precipitation occurs in a region of extreme turbulence and intense mixing, created by a jet of reactant liquid (e.g. drug solution) and a jet of another reactant liquid (e.g. antisolvent) coming through two facing nozzles mounted in a small chamber (Figure 4.3) [1]. As the two liquid jets mix, they react and cause the drug to precipitate as fine particles. The crucial process parameters include the speed of the liquid jets and the concentration of the reactants. A high jetstream speed or a high drug concentration have been found to give finer particles but a higher residual solvent level, while a low speed or low concentration produce the opposite effect [1]. The volume ratio of the reactants is also expected to affect the precipitation process.
Figure 4.3 Schematic diagram of the opposing impinging liquid-jet process. Reproduced with permission from Chan H-K, Kwok PCL. Production methods for nanodrug particles using the bottom-up approach. Advanced Drug Delivery Reviews 2011;63:406–416.
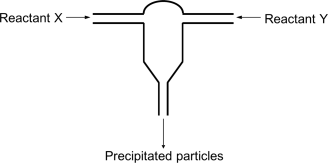
A requirement for the operation of the opposing-liquid-jets process is that the jets must have equal velocity, otherwise one jet will be pushed back by the other. This can severely limit the flexibility of the process. To get around this problem, the process has been modified to form a four-jet swirling configuration for enhanced mixing (Figure 4.4).
Figure 4.4 Schematic diagram of the four-jet swirling process. Reproduced with permission from Chan H-K, Kwok PCL. Production methods for nanodrug particles using the bottom-up approach. Advanced Drug Delivery Reviews 2011;63:406–416.
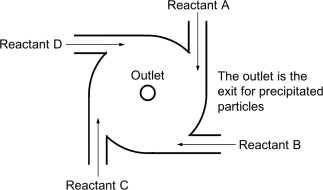
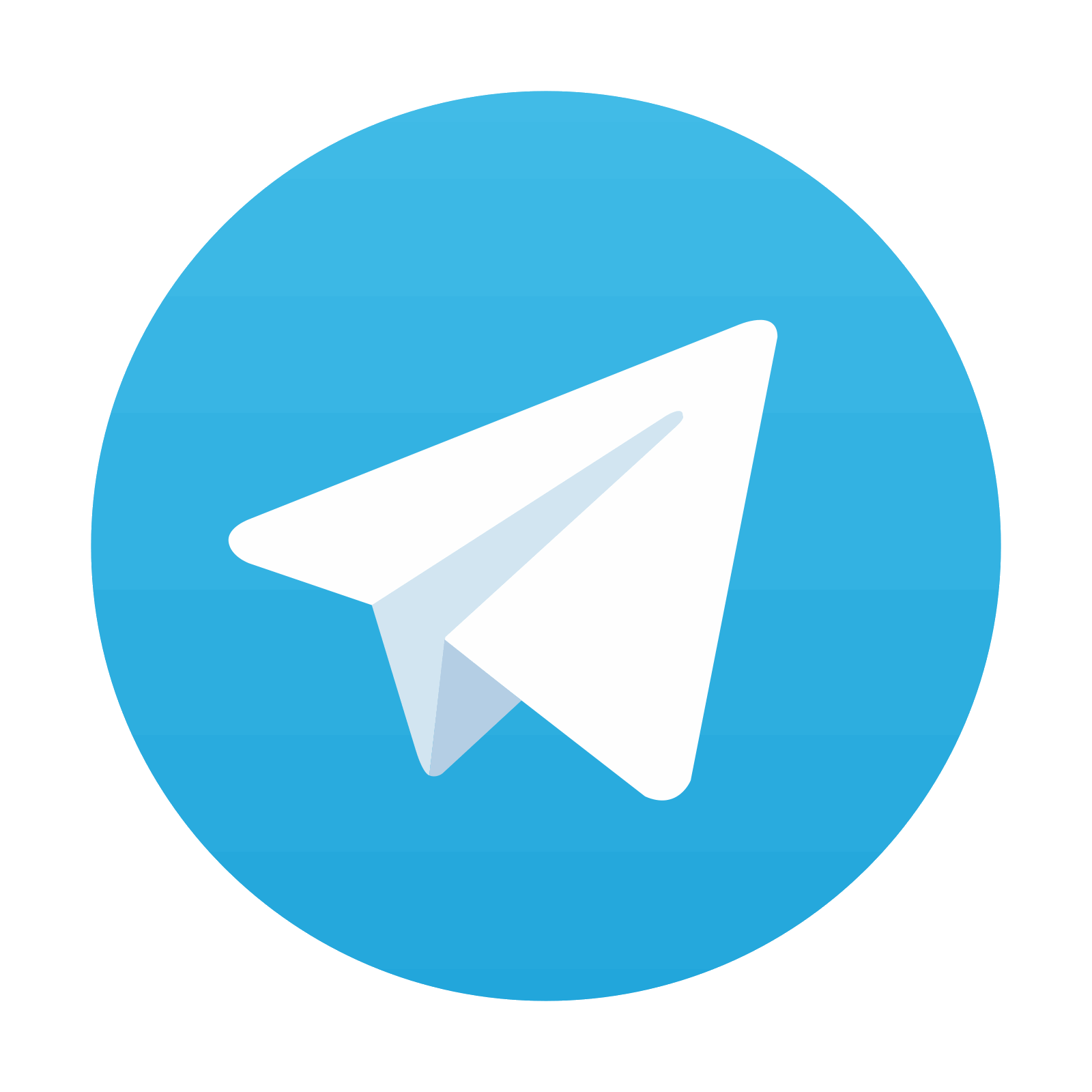
Stay updated, free articles. Join our Telegram channel
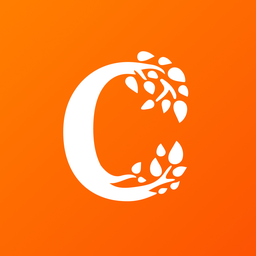
Full access? Get Clinical Tree
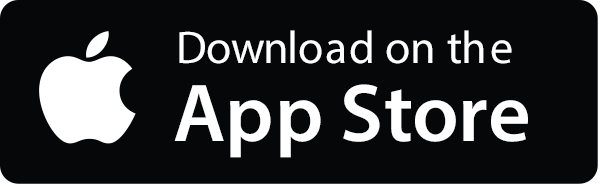
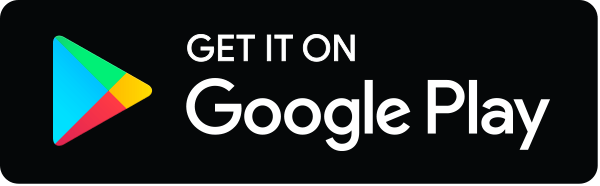