Acknowledgment
The writing of this chapter was supported in part by funding of the following NIH grants: DA012498, DA003628, and DA06634 (SEH).
Introduction
The comprehensive sequencing of human and other important genomes has enhanced our understanding of the cellular organization and function in higher organisms. This has been largely accomplished by the innovations in large-scale analysis of messenger RNA (mRNA) expression (microarrays, serial-analysis of gene expression [SAGE], and differential display). Genomics-based approaches have led to unprecedented advances in our understanding of the biological basis of substance abuse; however, the next step in systems biology is the examination of coordinate expression of the entire complement of proteins, including modifications and protein-protein interactions—proteomics. The broad-scale analysis of proteins in health and disease is essential given that proteins are central components of cellular physiology carrying out the greater part of biological events in the cell, even though certain mRNAs can act as effector molecules. Furthermore, it is important to note that mRNA and protein analyses are not interchangeable, with each being governed by distinct spatial, temporal, and physiological processes that generally prevent correlation of mRNA and protein expression in neuronal systems.
Proteomics involves the evaluation of the entire complement of proteins in a biological system with respect to structure, expression level, protein-protein interactions, posttranslational modifications (PTMs)—often referred to as structural, functional, and expression proteomics, respectively. The majority of early efforts in proteomics have been directed toward a comparison of differential protein expression and identification in disease and control tissues. However, changes in protein abundance do not define protein function exclusively, as many vital functions are brought about by PTMs, interactions among proteins, and differential distribution in subcellular components. Multiple proteomic strategies are needed to capture the involvement of regulatory mechanisms that affect protein abundance and function, such as protein-protein interactions and subcellular distribution.
The advent of proteomics can be attributed in part to the rapid development of mass spectrometry (MS), bioinformatics, and the current accessibility of vast protein databases from various organisms. These rapid advancements have improved our understanding of the cellular structure and function within the brain and the roles of various proteins and protein interactions in health and disease. However, the central nervous system poses unique challenges to proteomic inquiries, including the temporal and spatial expression characteristics of neurons and glia, the cellular heterogeneity of brain regions, the connectivity and communication between neurons, and the dynamic structural and functional alterations in neurons and glia that occur as a function of the interaction between the organism and the environment, development, learning and memory, and disease. These challenges can be overcome to some extent by combining specific isolation and fractionation procedures with high-throughput protein separation and analysis strategies to yield a more global view of the proteome in different physiological states than has been available previously. For example, before the advent of high-throughput proteomics technologies, our knowledge of protein alterations and the durations of those alterations induced by substance abuse was limited to fewer than 100 proteins—primarily expression levels of proteins assessed either individually or a few proteins at a time. With the development of proteomic technologies and strategies, it is now possible to evaluate significant portions of the neuroproteome (thousands of proteins) from crude homogenates to discrete cellular domains. Proteomic analysis strategies allow the simultaneous assessment of thousands of proteins of known and unknown function, thereby enabling a more comprehensive view of the protein orchestration in addictive disorders. Broad-scale evaluations of protein expression are well suited to the study of drug abuse, particularly in light of the complexity of the brain compared with other tissues, the multigenic nature of drug addiction, the vast representation of expressed proteins in the brain, and our relatively limited knowledge of the molecular pathology of this illness.
The development of innovative strategies has been ongoing in neuroproteomics, in particular for the study of PTMs, mapping of proteins from multiprotein complexes, and mapping of organelle proteomes. An understanding of the proteins in neurons along with their expression levels, their PTMs, as well as the protein-protein interaction maps would revolutionize addiction biology and addiction medicine in that we would then be able to expand our knowledge of the biochemical alterations specifically associated with substance abuse. Such information would be used to identify new targets for medication development.
Technology and Methods for Expression Proteomics
Protein Fractionation
The biological samples subjected to proteomic analysis in neuroscience include tissue, distinct cell populations, and cerebrospinal fluid (CSF). Each type of sample is extremely complex, as the protein constituents vary in charge, molecular mass, hydrophobicity, PTM, as well as spatial and temporal expression. The number of coding genes for the CNS fluctuate between 25,000 and 30,000. This added complexity of the neuroproteome will be overwhelming if we hypothesize that each protein on average has 10 splice variants, cleavage products, and PTMs, yielding approximately 250,000 to 300,000 protein isoforms to assess. Currently, there are no proteomic methods that have the capacity to separate and identify the entire proteome. One approach is to reduce the complexity of the proteome by subcellular fractionation procedures, thereby allowing a more thorough assessment of cellular domains (e.g., synapse, membrane, nucleus, and cytoplasm) while enriching low-abundance proteins that may not be detectable at the level of whole cell protein analysis.
Protein stability and purity as well as prevention of protein degradation and modification are of critical importance throughout various stages of proteomic analysis. Rapid removal of brain tissue, dissection, and freezing are imperative for the maintenance of the proteome state in the sample. Protease and phosphatase inhibitors are used to help prevent degradation and dephosphorylation of proteins during protein preparation ; however, care should be taken that adducts and charge trains are not introduced by these inhibitors. Purification of proteins from other cellular substances is also necessary, for example, lipids, several proteins (e.g., albumin and immunoglobulin are particularly abundant in the brain), and nucleic acids should be eliminated from the protein sample. The most common methods of purification rely on selective precipitation including acetone and trichloroacetic acid, although a number of commercially available kits are available.
Cerebrospinal Fluid
CSF is secreted by the choroid plexus in the lateral ventricles and is found in the cerebral ventricles and in the subarachnoid space flowing down the spinal canal as well as upward over the brain convexities. CSF, which an important determinant of the extracellular fluid (ECF) surrounding neurons and glia in the CNS, removes harmful brain metabolites, provides a mechanical cushion, and serves as a conduit for peptide hormones secreted by the hypothalamus. CSF is in steady state with the ECF and thus is considered to contain biochemical constituents that reflect neural activity.
Although proteomic studies of neuronal tissue have multiple challenges including the use of postmortem tissue and invasive biopsies from antemortem tissues, CSF proteomics is amenable to serial analysis by minimally invasive lumbar puncture. A change in the expression of CSF constituents may provide important insights into various CNS diseases by improving our understanding of the molecular basis of disease as well as providing disease biomarkers. Given the low protein concentration (∼150–450 μg/mL) and the high salt concentration (>150 mmol/L) of CSF and the abundance of albumin (∼60% of the total CSF protein) and immunoglobulin, it is necessary to deplete these abundant proteins (e.g., affinity removal, solid phase extraction) and reduce the salt concentration (e.g., protein affinity columns, ultrafiltration, and dialysis) to improve protein recovery and allow better detection of low-abundance proteins. This limitation, the depletion of some of the proteins of interest, can be overcome by a separate analysis of the depleted abundant proteins to ensure the analysis of proteins interacting with the abundant proteins.
Cellular Domain
Several recent proteomics studies have employed fractionation methods that allow collection of multiple cellular components from one tissue source. This allows a greater amount of each fraction to be used initially, thereby enabling analysis of low-abundance proteins. As the fractions are generated from the same samples, the experimental variability is reduced with the additional advantage of an additive increase in the whole proteome analyzed. The crucial drawback has been the overlap of the proteins between fractions.
Cytoplasm
Because the current proteomic strategies rely heavily on two-dimensional (2D) gel electrophoresis, which has been optimized for the analysis of soluble protein fractions, it is not surprising that most initial phases of proteomic analysis have focused on profiling of the cytoplasm. Most of the key regulators of the signaling pathways are housed in the cytoplasm, where, beside regulating the expression of receptors, they also channel important cytodynamic information between the nucleus and the membrane proteins. Some of the recent studies profiling the cytoplasm have revealed interesting new paradigms in our understanding of neurobiology.
Nucleus
The nucleus has a high degree of organization, consisting of structurally and functionally distinct compartments: nucleolus, nuclear speckles, nuclear pore complex, and nuclear envelope. The nucleus is a highly organized organelle consisting of domains that are fundamental for preserving the homeostasis of the cellular milieu. The profiling of the nuclear proteome in neuroscience has been the slowest of all subcellular fractions. However, there have been some good studies documenting the need to do so. In addition to the soluble fraction of nucleus, there has been an interest in other compartments of nucleus—nuclear envelope, nuclear pore complex, and nucleolus—although no studies to date have been published using such methods in addiction biology research.
Mitochondria
The mitochondria is a complex structure involved in fundamental processes, such as the tricarboxylic acid (TCA) cycle, β-oxidation of fatty acids, the urea cycle, electron transport, oxidative phosphorylation, apoptosis, and heme synthesis. Neuroproteomic analyses of the mitochondria have focused on the abundance in different brain regions. Data sets from mitochondrial proteomes from different species and tissues have documented 400–700 mitochondrial-associated proteins, which will enable scientists to better understand the mitochondrial machinery in health and disease.
Membrane
Membrane and the membrane-associated proteins constitute nearly a third of the cellular proteins and represent targets of approximately two-thirds of pharmaceutical agents. These proteins are involved in various cellular processes including signal transduction, cell adhesion, exocytosis, and metabolism and ion transport. Because membrane proteins are amphipathic, their hydrophobic nature makes them difficult to study and necessitates different strategies for analysis as compared to cytosolic proteins, for example. Therefore, although great strides have been made toward the analysis of soluble cellular proteins, the analysis of membrane proteins reported in proteomic analyses has been underrepresented. The traditional proteomic approach of two-dimensional gel electrophoresis (2DGE) has many limitations for analyzing membrane proteins —including the insolubility of hydrophobic proteins in a nondetergent sample buffer and inadequate alkaline-protein resolution. To a large extent, these issues can be overcome using a variety of combinations of liquid chromatographic separation techniques.
Synaptosomes and Postsynaptic Density
Synapses can be fractionated into synaptosomes as well as distinct pre- and postsynaptic components. Synaptosomes constitute the entire presynaptic terminal (including mitochondria and synaptic vesicles) and portions of the postsynaptic terminal (including postsynaptic membrane and postsynaptic density [PSD]). The study of synaptic proteomes is an important starting point in neuroscience for understanding complex brain functions, critical for understanding neuroplasticity as well as the neuropathology associated with drugs of abuse.
Synaptosomes are subcellular membranous structures formed during mild disruption of brain tissue. The shearing forces cause the nerve endings to break off and subsequent resealing of the membranes form the synaptosomes. The synaptosomes have a complex structure equipped with components of signal transduction, metabolic pathways, and organelles as well as structural components required for vesicular transport. Synaptosomes can be isolated from brain homogenate by differential and density-gradient centrifugation.
The postsynaptic density (or PSD) is a disk-like structure with a thickness of ∼30–40 nm and width of ∼100–200 nm. The most important structures associated with it are the cytoskeletal proteins, regulatory enzymes, and neurotransmitter receptors and associated proteins. These constitute a very highly structured framework with a definite association of the receptors and ion channels with the signaling molecules and the cytoskeletal elements to play an imperative role in signal transduction as well as synaptic plasticity. There are several available fractionation methods for isolation of the PSD.
Separation
Gel-Based Methods
Expression proteomics refers to the determination of protein levels without regard to PTMs. Gel-based as well as chromatographic separation approaches have been integral in generating proteomic profiles in numerous tissues including brain; however, research into the neuroproteome to date has been predominantly gel based.
Two-Dimensional Gel Electrophoresis
The basic principles of 2DGE remain the same since its introduction, namely the separation of proteins by isoelectric focusing ( IEF; first dimension) followed by sodium dodecyl sulfate polyacrylamide gel electrophoresis (SDS-PAGE; second dimension), which involves the separation of proteins by their molecular weight. In standard 2DGE experiments, approximately 1000–2000 proteins spots are visualized on a gel representing the most abundant proteins, while other low-abundance proteins are largely obscured by the more abundant proteins. Subcellular fractionation can be used to enrich the representation of low-abundance proteins. Caveats of the 2DGE procedure include (1) the possibility of comigration of proteins (i.e., many proteins in a spot); (2) migration of proteins as multiple spots (i.e., due to charge trains, PTMs, isoforms, and so on); (3) intensive image analysis requiring manual removal of artifacts; (4) inability or difficulty of large and hydrophobic proteins to be isolated in first-dimension gels; and (5) poor representation of highly acidic and basic proteins (i.e., membrane-bound proteins). In general, 2DGE variability is approximately 20%–30% due to sample preparation, reagent sources, staining methods, image analysis software, and technical expertise and experience.
Isoelectric Focusing
Following protein solubilization, the next step in 2DGE is isoelectric focusing (or IEF), which separates the proteins in the first dimension according to their isoelectric point (or pI). The pI of a protein is primarily a function of the amino acid side chains that are protonated or deprotonated, depending on the pH of the solution in which the protein is present. For IEF, protein samples are loaded onto strip gels consisting of a gradient of pH values, and electrophoresis leads protein migration depending on the net charge of each protein in the sample. At a specific pI, the protein will reach the point in the pH gradient where the net charge of the protein is zero and stop migrating.
SDS-PAGE
Next, the IEF gel or strip is equilibrated with sodium dodecyl sulfate (SDS) and placed on top of the SDS acrylamide gel. The equilibration step is necessary to allow the SDS molecules to complex with the proteins and produce anionic complexes with net negative charge roughly equivalent to the molecular weight of the protein. Proteins are electrophoresed migrating from the IEF gel and into the SDS gel, where they separate according to molecular weight (second dimension). Both conventional SDS-PAGE instruments, such as those used for western blotting, and special purpose apparatuses can be used for this step.
Gel Staining
Following electrophoresis, it is imperative to visualize gel spots for subsequent isolation and MS analysis. Coomassie Brilliant Blue (CBB), silver nitrate, and negative staining are common postelectrophoresis methods available for the 2D gel-based proteomics analysis. The sensitivity of these stains range from 100 ng (e.g., CBB) to 1 ng (e.g., silver nitrate) for individual protein spot detection. In acidic medium, CBB binds to the amino acids by electrostatic and hydrophobic interactions; however, some of the proteins release the dye during the destaining procedure, which may cause problems with reproducibility and quantitative reliability. CBB is compatible with MS, as complete destaining of the gel can be achieved using bicarbonate. As a rule of thumb, proteins detected visually by CBB are sufficiently abundant for characterization by MS. Disadvantages of CBB staining include low sensitivity and a narrow dynamic range, which however is better than silver stain. Silver staining is widely used for quantitative analysis due to its high sensitivity. Despite its excellent sensitivity, silver staining lacks reproducibility, has a limited linear dynamic range, subjective judgment of the staining end-point, and interferes with the MS compatibility, resulting in a much lower sequence coverage compared to CBB. Even though silver staining is still used currently, there has been an increasing trend to use the new-generation fluorescent stains.
Fluorescence-based detection methods are more sensitive than the absorbance-based methods given the difference in detected and incident wavelengths, which lead to lower background values. SyproRuby dye (Molecular Probes, Eugene, OR), the first of the fluorescent stains, is part of a stable organic complex composed of ruthenium, which interacts noncovalently with basic amino acids in proteins. The stain can be visualized using a wide range of excitation sources commonly used in the image analysis systems. It has a sensitivity that approximates silver staining with a linear dynamic range of three orders of magnitude. DeepPurple (GE Healthcare, Piscataway, NJ) possesses a broad dynamic detection range over four orders of magnitude with limited speckling and background staining, appears to result in increased peptide recovery from in-gel digests compared to SyproRuby stain and improves matrix-assisted laser desorption/ionization time of flight (MALDI-ToF) MS-based identification of lower abundance protein spots by increasing sequence coverage.
Two-Dimensional Difference in Gel Electrophoresis
Whereas 2DGE has been the workhorse of proteomics for several decades, the method has been plagued by issues of reproducibility and quantitation given that multiple gels have to be compared. Two-dimensional difference in gel electrophoresis (2D-DIGE) allows the labeling of two to three samples with different dyes on the same 2D gel, thereby reducing spot-pattern variability and the number of gels in an experiment—with the result of making spot matching much more simple and accurate. The most popularized experimental design has been the use of a pooled internal standard (sample composed of equal aliquots of each sample in the experiment) labeled with the Cy2 dye and labeling control and experimental samples with Cy3 or Cy5 dyes swapped equally across the samples, respectively. Following first- and second-dimension electrophoresis, gels are sequentially scanned for Cy2-, Cy3-, and Cy5-labeled proteins by the following lasers/emission filters; 488-/520-, 532-/580-, and 633-nm/670-nm, respectively. The scanned images of the fluorescence-labeled proteins are sequentially analyzed by differential in-gel analysis (DIA; performs Cy5/Cy3: Cy2 normalization) followed by biological variation analysis (BVA; performs inter-gel statistical analysis to provide relative abundance in various groups). These log abundance ratios are then compared between the control and diseased/treatment samples from all the gels using statistical analysis ( t test and analysis of variance [ANOVA]).
A modification of 2D-DIGE in which cyanine dyes that label all of the cysteine residues of proteins has been introduced with a detection limit for saturation labeling of 0.1 ng protein per spot thereby reducing the amount of protein sample required for analysis. This procedure provides a very attractive alternative for performing quantitative 2D-DIGE when dealing with low sample amounts, typical in neuroscience, even though only two saturation dyes are currently available (Cy3 and Cy5).
Chromatographic Separation of Proteins
The coupling of efficient chromatographic and electrophoretic separation methods with high-performance MS holds great promise for qualitative and quantitative characterization of highly complex protein mixtures. The advances in chemical tagging and isotope-labeling techniques have enabled the quantitative analysis of proteomes. Multidimensional liquid chromatographic separation (also known as multidimensional protein identification technology; MudPIT ) is typically based on using two or more physical properties of peptides (size, charge, hydrophobicity, and affinity) to reduce the complexity of the proteome. Methods commonly used to separate peptides based on physical and chemical properties include ultracentrifugation (density), capillary electrophoresis (size and charge), isoelectric focusing (isoelectric point), size-exclusion chromatography (Stoke’s radius), ion-exchange chromatography (charge), hydrophobic interaction chromatography (hydrophobicity), reverse-phase chromatography (hydrophobicity), and affinity chromatography (biomolecular interactions).
A major advantage of multidimensional approaches over 2DGE methods is the ability to isolate low-abundance proteins as well as the proteins with extreme pI, molecular weight, and hydrophobicity. In most multidimensional separation approaches, proteins are digested into peptides prior to separation yielding complex peptide mixtures but with increased solubility due to the elimination of nonsoluble hydrophobic peptides—a critical caveat for the study of membrane proteins that are insoluble in aqueous buffers.
Several strategies have been developed for relative quantitation of protein expression between samples including (1) isotopic labeling of separate protein mixtures, (2) combined digestion of the labeled proteins followed by multidimensional liquid chromatographic separation, (3) automated tandem mass spectroscopy (MS/MS) of the separated peptides, and (4) automated database search to identify the peptide sequences and quantify the relative protein abundance based on the MS/MS.
Isotope-Coded Affinity Tags (ICAT and iTRAQ)
Isotope-coded affinity tags (ICAT) used to be is one of the most popular methods for quantitative proteome analysis before the inception of isobaric tags for relative and absolute quantitation (iTRAQ) multiplex quantitation strategy. The ICAT reagent is composed of a cysteine-reactive group, a linker containing the heavy or light isotopes (d8/d0), and a biotin affinity tag. The labeling method involves in vitro derivatization of cysteine residues in a protein with d0 or d8 followed by enzymatic digestion of the combined sample. All cysteine biotin-tagged residues are selectively separated by avidin column followed by further separation using reverse-phase chromatography. The isotopically tagged peptides give quantitative mass spectrometry analysis based on the relative peak intensities/areas of d0 and d8 labeled peptides. Another advantage is the ability to analyze peptides with molecular weight more than 3000 Da easily because the mass difference between the coded isoforms is sufficiently large.
A major limitation of ICAT is the exclusive analysis of cysteine containing peptides (10%–20% of the peptides). The resolution is greatest in the case of smaller peptides where the d8/d0 ratio is higher and with peptides that have multiple cysteine residues. Another limitation is that the biotin affinity tag remains linked to the peptides throughout the analysis, causing shifts in chromatographic separation, shifts in the mass-to-charge ratio (m/z), and changes to MS/MS spectra relative to the unlabeled peptides complicating the manual or computer-assisted interpretation. Most analyses of ICAT have utilized the combination of strong cation exchange (SCX) chromatography with reverse-phase microbore liquid chromatography coupled with on-line (RP-μLC) with MS and MS/MS. Data-dependent software is used to select specific mass/charge (m/z) peptides for CID, alternating MS and MS/MS scans for collecting qualitative and quantitative data. Alternative strategies such as per-methyl esterification of carboxylic acid groups ,18 specific labeling of lysine residues, and peptide N -termini have also been used recently. Quantification software has been developed, which can assemble a composite ratio for a protein based on the calculated expression ratio from all the peptides from a single protein such as XPRESS ( http://tools.proteomecenter.org/XPRESS.php ) and ProICAT (Applied Biosystems, Foster City, CA) The data obtained from these applications can be analyzed collectively using INTERACT for multiple experiments.
iTRAQ methodology is an extension of ICAT, which uses four isobaric reagents (114, 115, 116, and 117), allowing the multiplexing of four different samples in a single LC-MS/MS experiment. More recently, iTRAQ 8Plex, which has four more isobaric reagents (113, 118, 119, and 121) in addition to the traditional four iTRAQ reagents, expands the possibilities of using more experimental variables for comparison. A major advantage of this technique over the ICAT is the ability to label multiple peptides per protein; this increases the confidence of identification as well as quantitation. A recent study comparing 2 DGE and iTRAQ reported a confidence interval of 0.24 for isobaric tagging versus 0.31 for 2 DGE as well as a greater range of expression ratios. A more recent study compared 2D-DIGE, ICAT, and iTRAQ and reported that iTRAQ was more sensitive than the ICAT, which was equisensitive to 2D-DIGE. The complementary nature of these techniques was confirmed by the limited overlap of the proteins characterized.
Top-Down Proteomics
The aforementioned techniques (bottom-up proteomics) are based on consistent enzymatic conversion of proteins to peptides. It is customary to accurately make mass measurements by MS/MS of lower molecular-weight peptides rather than higher molecular-weight intact proteins; however, the bottom-up approach increases the sample complexity, and the entire sequence coverage for proteins is rarely achieved, thereby limiting site-specific PTM analysis of proteins. Such limitations have renewed interest in top-down proteome characterization strategies. Such techniques characterize individual proteins by MS without prior enzymatic cleavage. Capillary isoelectric focusing (CIEF) coupled with Fourier transform-ion cyclotron resonance (FTICR) MS is one such strategy for analyzing complex protein mixtures using a top-down approach. One potential major limitation is that the level of information is not always sufficient for confident protein identification due to the possibilities of point mutations, PTMs, and the presence of open reading frames having high-sequence homology. This problem can be overcome somewhat by incorporation of isotopically labeled amino acids into the cellular proteins of unicellular model organisms. The partial amino acid content information obtained combined with CIEF-FTICR, enables identification of proteins from genome databases without MS/MS information. Other limitations include the large amount of sample required and the low-throughput that is not amenable to automation.
Mass Spectrometry
Mass spectrometers consist of three major units: the ion source, the mass analyzer, and the ion detection system. MS is based on the separation of ionized proteins or peptides according to the mass to charge ratio (m/z). Tandem mass spectrometry (or MS/MS), on the other hand, couples two mass spectrometers in time and space and has revolutionized the field of expression and functional proteomics. MS/MS involves selection of peptides of a certain mass and the subsequent fragmentation and mass analysis (in two stages). In the first stage, the precursor ion produced by the ion source is selected for fragmentation. The fragmentation results in production of product ions to be analyzed in the second stage of mass analysis. The inconvertible link between the precursor ion and the product ions is responsible for the unique molecular specificity of MS/MS.
Ion Source
A number of ionization technologies exist including fast ion bombardment (FAB), matrix-assisted laser desorption ionization (MALDI), and electrospray ionization (ESI). MALDI and ESI are the techniques of choice for most proteomic applications of neuroscience research. MALDI works by mixing the protein sample with a light-absorbing matrix that forms a crystal. This is usually done on some form of plate with multiple positions for different samples. When the plate is pulsed with a laser of a particular wavelength, the energy from the laser is absorbed by the crystal matrix and the proteins within the crystal are ionized and desorbed (ejected) from the plate into the mass analyzer.
In ESI (and nanospray ionization), ions are produced in a liquid phase. The protein sample, in a solvent solution, is ejected as a mist of droplets from a charged capillary tip. As the solvent in the droplets evaporates, the total charges of the proteins in the droplet remain but with a reduced surface area of the droplet. This continues to a point at which individual ions leave the droplet. Individual ions then pass on into the mass analyzer.
Mass Analyzers
Whichever method of ionization is used, once the ions are created they must be separated before being detected in such a way as to provide information on the m/z ratio. Mass analyzers do not actually detect the ions or measure ion mass; they are used only to separate ions according to their m/z ratio. A number of mass analyzer types exist: time-of-flight (ToF), quadrapole, ion trap, and Fourier transform ion cyclotron resonance (or FTICR).
ToF mass analyzers can be thought of as a tube. The ionized proteins enter the tube by passing through a high-voltage accelerator. The speed at which the ion travels is proportional to its mass (m). The number of ions are produced simultaneously and pass through the ToF tube and to a detector; the ions with a higher m/z ratio will travel faster and reach the detector first. Since the distance traveled and time are all known, the m/z ratio can be calculated and from that the mass.
Quadrapole mass analyzers also involve ions traveling down what can be thought of as a tube. In this case though, the tube consists of four parallel rods. The rods are two pairs of two that can be tuned to different currents and radio frequencies. The two pairs of rods have opposite currents and shifted radio frequencies allowing a form of tuning in which only ions of a particular m/z ratio pass though the tube. A range of m/z ratios can be scanned, generating an m/z profile of the sample. Quadrapole mass analyzers are often used with an ESI ion source.
Ion trap mass analyzers use the same principles as the quadrapole in that specific combinations of current and radio frequencies are used to select particular m/z ratios. The ion trap can be thought of as a small ball with one electrode around the equator and two more electrodes at the poles. Ions are introduced into the center of the ball and are kept in orbits within the trap. By changing current and radio frequency combinations, particular m/z ratio ions are ejected from the ion trap through a port to the detector. By scanning though these voltages and radio frequencies a complete m/z profile can made.
A number of hybrids of these separation strategies exist, all of which are generally designed to increase the accuracy of m/z measurements and sensitivity to low-abundance ions. ToF analyzers can be placed in series (ToF/ToF) with a reflectron or collision cell between them, quadrupoles and ToF can be placed in series (Q-ToF), and extremely powerful magnets and Fourier transform algorithms (FTICR) can be used to determine the m/z ratios of all ions within an ion trap. Detectors change the kinetic energy of the ions into an electrical current that can be measured and passed along to a computer. Although these detectors give information on the abundance of ions, quantitation of protein abundance differences between samples by MS is limited unless samples are linked to isotopes (see ICAT).
All of these MS techniques can be applied to complex protein samples, that is, a mixture of hundreds or thousands of proteins. It is important to separate the use of MS instruments to separate proteins from the MS used for protein identification, as will be described later. As described in subsequent text, quantitative analysis by MS is limited to techniques like ICAT. For researchers looking to profile the expression of proteins in a large number of samples, MS can be problematic and requires a great deal of time on expensive instruments.
Protein Identification
No matter the separation and quantitation methods used, at the end of the experiment the proteins must be identified. Most approaches use mass spectrometry. Peptide mass fingerprinting (PMF) and tandem mass spectrometry (or MS/MS) are the main methods for determining protein identities. PMF was developed by a number of research groups and begins with digestion of a protein with an enzyme, typically trypsin. Trypsin cleaves proteins at very specific locations, resulting in a series of peptides. If this mixture of peptides is analyzed by MS, a series of peptide masses is created. These masses are searched against databases using one of a number of programs (e.g., ProFound and MASCOT). These programs take DNA sequence databases translated into protein sequences and calculate the resulting peptide masses if these protein sequences were digested with trypsin. The peptide masses generated from the MS of the digested protein of interest is then compared against these databases and the protein can be identified. PMF of spots from 2DGE gels is one very common application. Gel plugs are either excised by hand or robot. These plugs contain the proteins of interest and the proteins are digested in the plugs with trypsin. With visual stains, the plug must often be destained, and some stains work better than others. Silver stains that use glutaraldehyde are not compatible with MS.
Even if MS instead of 2DGE was chosen as the method of protein separation, MS is also used for protein identification through a process called tandem mass spectrometry (or MS/MS). A number of different strategies exist for MS/MS; in general the process entails the selection of one ion/peptide generated during initial MS and then fragmenting this ion/peptide into smaller pieces and measuring the mass of the resulting ions. These secondary ions can be decoded into peptide sequence information that can be searched against protein sequence databases to identify the protein. Almost all of the ionization and mass analyzer types can all be used for MS/MS, provided that the instrument is appropriately configured. One MS/MS method that is particularly suited for proteome determination, but less so for quantitation, is multidimensional protein identification technology (or MudPIT). In this method all the proteins in a sample are digested and loaded onto LC columns (see previous explanation). After fractionation of the peptides, the peptides are fed into an MS/MS instrument for protein identification. This method has identified thousands of proteins, can detect membrane proteins, and is similar in concept to shotgun sequencing of DNA.
Some of the more traditional methods for identifying proteins are still used for proteomic experiments. Edman protein sequencing can be performed on proteins or peptides extracted from gels or blotted from gels, although the method is limited by low throughput and requires a comparatively large amount of protein. Another technique is the far western blot, where a 2DGE gel is blotted and probed with an antibody against a specific protein. This approach does not offer much progress over conventional immunoblotting.
Protein Arrays
Protein arrays provide a means to characterize the function, interactions, and activities of a large number of target proteins. Protein arrays are generally divided into three categories: antibody (analytical protein) arrays, functional protein arrays, and reverse-phase protein arrays Antibody arrays utilize either antibody capture of labeled proteins or the sandwich-based immunoassay in which the protein of interest is captured by one antibody adhered to the array surface and a second antibody that is labeled for detection. Antibody arrays are probably most applicable in addiction medicine as a means of quantifying protein levels in various tissues and fluids as well as a rapid assay for potential biomarkers. Functional protein arrays are useful for determining the biochemical properties of proteins including protein binding and enzyme-substrate interactions. Arrays are produced by adhering purified proteins to the array surface and probing with labeled proteins or substrates. This type of array is used to study the following types of functional protein interactions: protein/protein, protein/peptide, protein/DNA, protein/RNA, protein/lipid, protein/small molecule, and protein/glycan.
Due to some of the limitations of electrophoresis and MS methods, selected research groups are attempting to create proteomic chips/arrays. Antibodies or other affinity reagents (e.g., aptamers, peptides) are spotted onto some sort of matrix. Hundreds to thousands of spots are on a single array. A labeled sample is then washed across the array and proteins bind to their specific antibody. The process can also be reversed whereby the protein samples of interest are spotted onto the matrix and then probed with different affinity reagents. Although these array or chip approaches have potential for greatly increasing the throughput of proteomic experiments, the use of affinity reagents as the separation method is a severely limiting factor and cannot be ignored. A high-quality antibody is needed for each protein of interest and each modification of that protein. To generate quantitative data from antibody arrays, and because association kinetics between different antibodies and antigens can vary tremendously, relative concentrations of each antibody and antigen have to optimized for each protein. Although there seem to be a number of pitfalls to proteomic chips/arrays as an open-screen technique, they do hold promise for routine examination of a small group of proteins. Well-known pathways or gene families could be easily examined by such an approach.
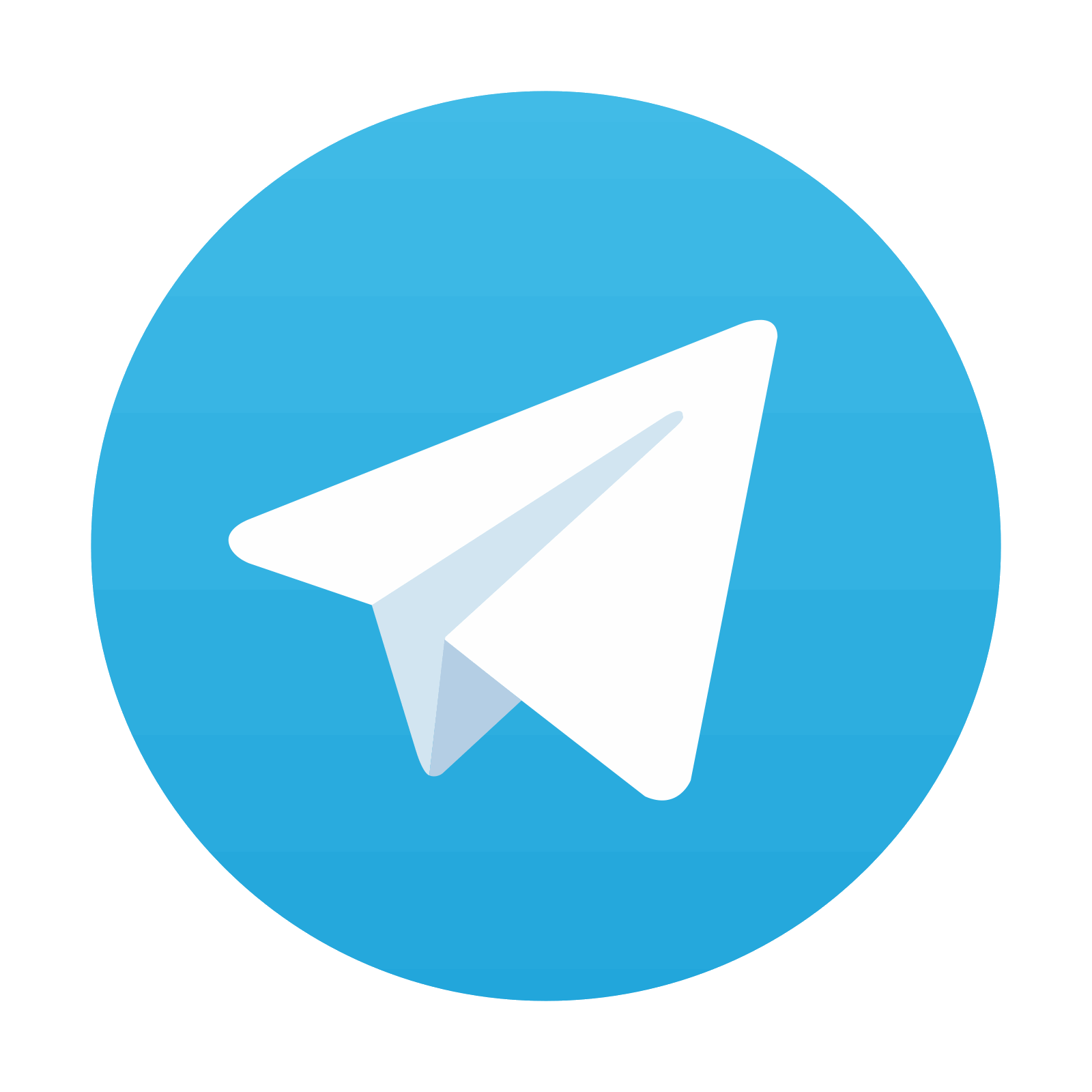
Stay updated, free articles. Join our Telegram channel
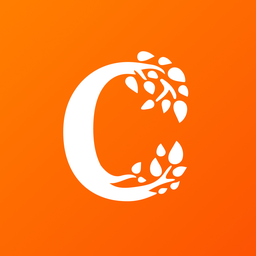
Full access? Get Clinical Tree
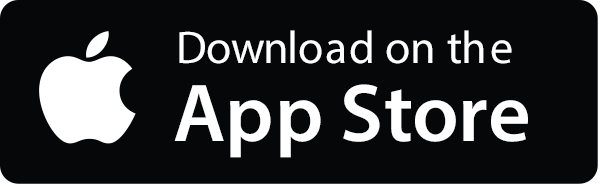
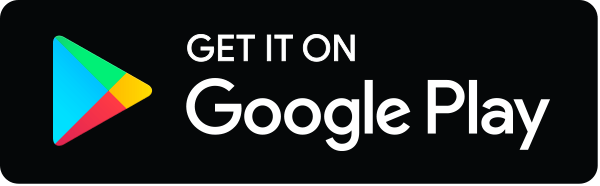