Chapter 7 Neuromuscular physiology
7.1 Relevant structure and organization of the nervous system
Subdivisions of the nervous system
An important distinction is made between the somatic nervous system, supplying the skin and musculoskeletal structures, and the autonomic nerves, which are distributed both to smooth muscle in the blood vessels and viscera of the body and to exocrine and certain endocrine glands.
Neuronal structure
The main functional cell in the nervous system is the nerve cell or neurone, which is primarily engaged in signalling and control. Classically, each neurone is described as having three elements, with the cell body or soma connected to a large number of short projections, known as dendrites, and a single elongated projection called an axon (Fig. 116). Incoming signals affect the dendrites and soma, while outgoing signals are passed on via the axon. The connection between one nerve cell and the next is known as a synapse and there is often a swelling at the nerve ending known as a synaptic bouton. The membranes of adjacent nerve cells do not come into direct contact with each other at these sites, however, but are separated by a synaptic cleft. This separation has important consequences for signal transmission between cells.
7.2 Conduction and transmission in nerves
Information is conducted along nerves in the form of ‘all or nothing’ electrical signals known as action potentials (Fig. 117A). Intracellular recordings from axons show that the inside is normally negative with respect to the outside, i.e., there is a resting membrane potential (about −70 mV). If a stimulus decreases this potential (i.e., makes it less negative), so that the membrane reaches the threshold potential, then there is further, automatic and rapid depolarization which reverses the potential across the cell membrane. This is an active event and is followed by repolarization to resting conditions. The properties of this action potential, and the membrane mechanisms which generate it, have been considered in detail in Section 1.4. Little more need be said here other than to remind ourselves that depolarization reflects a voltage-dependent increase in the membrane permeability, or conductance, to Na+, while repolarization is caused by the slower onset of a similar rise in K+ conductance.
Compound action potentials
An anatomically identifiable peripheral nerve, such as the sciatic nerve in the pelvis and thigh, is made up of a large number of individual axons. The extracellularly recorded electrical activity in such a nerve reflects the total effect of all the individual action potentials in different axons and is called a compound action potential. When such a nerve is stimulated electrically, it can be shown that the amplitude of the compound action potential increases as the stimulus strength is increased (Fig. 117B). This observation appears to contravene the ‘all or nothing’ law for excitable cells but, in fact, it simply reflects the presence of axons with differing thresholds within the nerve. As the stimulus is increased, more and more axons reach threshold so that a greater proportion of the available fibres fire an action potential. Eventually all the axons will have been recruited in this way and further increases in the stimulus produce no further change in the compound action potential. These are referred to as supramaximal stimuli.
Action potential conduction velocity
Once initiated, action potentials are propagated, or conducted along an axon by means of local currents (Section 1.4). Positive current spreads along the axon away from the site of an action potential and this depolarizes the adjacent membrane. Once threshold potential is reached, an action potential will be actively generated, and the whole process is repeated. Although the local currents are conducted equally in both directions along the axon, the fact that the nerve membrane remains refractory to further stimulation for a few milliseconds after firing an action potential ensures that the signal is conducted along the axon in one direction only.
Myelination
Conduction velocity is greatly increased in myelinated, as opposed to unmyelinated, nerves. Myelin sheaths are produced by Schwann cells in peripheral nerves and oligodendrocytes in the CNS. These glial cells wrap their plasma membranes round and round the axon to produce a sheath of tightly stacked bilipid layers (Fig. 118). This myelin is not continuous, however, and there are gaps in the sheath, known as nodes of Ranvier, spaced at regular intervals along the axon. Action potentials are only generated at these sites, where the excitable membrane of the neurone is exposed. The spread of depolarizing current along the myelinated region to the next node is very rapid and the electrical insulation provided by the lipid myelin sheath helps to promote this rapid conduction by reducing the leakage of local current across the nerve membrane, leaving more available to depolarize the axon at the nodes. This type of conduction, in which the action potential effectively jumps from one node to the next along the axon, is referred to as saltatory conduction and is considerably more rapid than continuous conduction in unmyelinated nerves. Myelinated nerves conduct with velocities in the range 10–120 m s−1, depending on their size, with the large somatic nerves to skeletal muscles conducting the fastest. Small, unmyelinated nerves, by comparison, may conduct at less than 1 m s−1, e.g., slow pain fibres and autonomic motor nerves.
Synaptic transmission
When an action potential reaches an axon ending, it must then influence the adjacent nerve if the signal is to be passed on. This occurs at the synapses between the axon of one neurone and the dendrites or cell body of the next (Fig. 116), and the process is referred to as synaptic transmission. Transmission usually depends on the release of a messenger molecule called a neurotransmitter from the nerve terminal, and this chemical affects the adjacent cell, tending either to excite or to inhibit action potential generation in that neurone. This is an example of chemical transmission.
Structure and function of the synapse
The nerve along which incoming signals arrive at the synapse is called the presynaptic nerve and this characteristically terminates in an expanded structure called a terminal bouton (Fig. 119A). Electron micrographs demonstrate that these boutons enclose a large number of membrane-bound vesicles containing neurotransmitter. A narrow gap (about 20 nm) known as the synaptic cleft separates the presynaptic membrane from that of the postsynaptic neurone. The existence of this separation between adjacent nerves suggests a chemical transmission mechanism, since electrical transmission (the possible alternative mode of transmission) requires electrical continuity between the cytoplasm of adjacent cells (e.g., the gap junctions between cardiac cells; Section 3.1).
The general sequence of events in synaptic transmission may be summarized as indicated by the numbered steps in Figure 119B.
Neurotransmitters
Acetylcholine is an important excitatory transmitter in the brain and spinal cord. It is also involved in ganglionic transmission in autonomic nerves, as well as being the peripheral transmitter at the skeletal neuromuscular junction (Section 7.5) and in postganglionic parasympathetic nerves (Section 7.6).
Biogenic amines include the catecholamines, 5-hydroxytryptamine (serotonin) and histamine. Catecholamines (dopamine, noradrenaline (norepinephrine) and adrenaline (epinephrine)) share a common synthetic pathway involving conversion of the amino acid tyrosine to L-dopa and then to dopamine (Fig. 120). This may then be further modified to form adrenaline (epinephrine) or noradrenaline (norepinephrine). After transmitter release, catecholamine action is terminated by reuptake into the neurones followed by breakdown through the action of monoamine oxidase (in the presynaptic neurone) or catechol-O-methyl transferase (on the postsynaptic membrane). Drugs which inhibit monoamine oxidase promote catecholamine-dependent transmission in the brain and are used in the treatment of clinical depression. Inadequate dopaminergic transmission is also believed to be involved in a motor disorder called Parkinson’s disease (Section 7.5) and treatment with the dopamine precursor L-dopa is sometimes beneficial, presumably because it increases dopamine synthesis. Conversely, drugs which inhibit dopaminergic transmission by blocking dopamine receptors are used in the treatment of schizophrenia, a psychiatric disorder characterized by disrupted thought, hallucinations and delusions. One side-effect of this treatment can be the development of Parkinsonian type symptoms, which is consistent with the idea that dopamine deficiency is involved in causing Parkinson’s disease.
Peptides
A wide range of peptides have now been identified as probable or possible neurotransmitters. Examples include substance P, which seems to play a role in the central transmission of pain signals, and endorphins, which inhibit pain pathways, perhaps by blocking release of substance P (Section 7.4). Some peptide transmitters may also be released along with a classical transmitter by a single neurone, e.g., acetylcholine and vasoactive intestinal polypeptide (VIP) coexist in some parasympathetic nerves. This phenomenon is known as cotransmission.
Excitatory transmission
Excitatory neurotransmitters act on the postsynaptic membrane to produce depolarization, thus favouring the firing of an action potential. The electrical response recorded from the postsynaptic neurone consists of a brief depolarization followed by a slower decline to the resting potential (Fig. 121), and is known as an excitatory postsynaptic potential (EPSP). This differs from the action potential in two main ways.
Graded response
The EPSP is a graded response, not an ‘all or nothing’ response like the action potential (Fig. 122A). The larger the number of excitatory nerves which are stimulated simultaneously, the larger is the resulting EPSP. This is called spatial summation, since the effects of excitatory neurotrans-mitter release at many different synapses on the postsynaptic neurone add together to give a larger total response. Similarly, repeated stimulation in a single excitatory nerve may increase the peak of the EPSP, since further depolarization occurs before the membrane returns to resting potential. This is temporal summation (Fig. 122B). If summation leads to a large enough depolarization (i.e., reaches threshold), the postsynaptic cell will generate an action potential which then travels down its axon.
Inhibitory transmission
The simplest form of inhibition (postsynaptic inhibition) is analogous to excitation in that synaptic transmission produces a change in postsynaptic membrane potential. In this case, however, hyperpolarization results (Fig. 123A), and this is termed the inhibitory postsynaptic potential (IPSP). By moving the membrane further away from the threshold potential, this makes it less likely that an action potential will be generated in response to other excitatory stimuli. In other words, IPSPs and EPSPs from different synapses acting on a given neurone will summate algebraically (Fig. 123B). The signals from the many different inputs to a cell are integrated in this way and an action potential will only be generated if the postsynaptic membrane in the region of the axon reaches threshold. Overall, the action potential frequency will reflect the balance between excitatory and inhibitory influences at any time.
Presynaptic inhibition
It is possible to reduce the size of the EPSP generated by excitatory stimulation through inhibitory nerves which do not produce any IPSP in the postsynaptic neurone. This presynaptic inhibition depends on inhibitory nerves which synapse with the incoming excitatory axon, rather than the postsynaptic cell itself (Fig. 124A). Activity in this inhibitory pathway reduces the amount of excitatory neurotransmitter released when the axon is stimulated. This depends on depolarization of the axon by the presynaptic neurotransmitter, which decreases the amplitude of any action potentials within that nerve terminal since the potential rises from an elevated baseline potential. The quantity of transmitter released is dependent on the size of the action potential and so the resulting EPSP is smaller than normal (Fig. 124B). Certain inhibitory interneurones in the spinal cord are believed to exert their influence on spinal motoneurones through presynaptic inhibition of this kind.
Ionic mechanisms of postsynaptic potentials
Neurotransmitters produce the changes in postsynaptic potential described in the preceding sections by opening receptor-operated ion channels. In the case of spinal motoneurones it seems likely that:
EPSPs are produced in response to the excitatory transmitter glutamate which opens channels allowing both Na+ and K+ to flow through them (nonselective cation channels). Overall, this increases the permeability of the membrane to Na+ relative to that to K+ and, since ENa (the equilibrium potential for sodium) is positive, this tends to depolarize the cell (Section 1.4).
7.3 General sensory mechanisms
Sensory physiology deals with the mechanisms involved in the detection and interpretation of a wide variety of different stimuli. This relies on sensory receptors to convert these stimuli into electrical signals within the sensory nerves, which relay the information to the CNS. Within the CNS the sensory inputs are used both to control the movements of the body and to regulate the internal environment (homeostasis). This requires storage of reference information about normal conditions, e.g., the set points for different controlled variables (Section 1.1), and appropriate connections between the sensory (afferent) and motor (efferent) pathways so that compensatory adjustments can be made. Conscious awareness and interpretation of sensation is also a central nervous function, with particular areas of the cerebral cortex being devoted to processing activity from specific types of receptor.
Receptor types
Receptors may be classified in terms of the general class of stimulus to which they respond.
Receptors may also be classified in terms of the purpose they serve. This is subtly different from a classification based on stimulus type. For example, nociceptors consist of all receptors responsible for painful sensations but this grouping contains a mixture of mechano-, thermo- and chemoreceptors (Section 7.4). Similarly, proprioceptors represent a specific subgroup of mechanoreceptors, all of which provide information about joint position.
Receptor potentials
Sensory receptors convert a stimulus into an electrical response known as a receptor potential. This is often a graded depolarization that increases in amplitude as the stimulus strength increases (Fig. 125). Receptor potentials are localized to the receptor region itself and are not actively propagated along the sensory nerve. However, if the receptor potential depolarizes the nerve to threshold level, it stimulates action potentials that are then conducted along the sensory axon to the spinal cord. This relies on passive depolarization of the axon in receptors formed by adaptation of a sensory nerve ending, e.g., mechanoreceptors in the skin. In other cases, e.g., the hair cells in the inner ear, the receptor cells may be distinct from the relevant sensory axons. Here, the receptor potential controls transmitter release from the receptor itself, and this depolarizes the sensory axon, producing a graded generator potential. If this reaches threshold, propagating sensory action potentials result.
Coding for stimulus strength in the sensory system
Thus, increasing stimulus strength produces larger receptor potentials which generate a higher frequency of action potentials in the sensory axon (Fig. 125).
Sensory adaptation
If a constant stimulus is applied continuously to a receptor, the resulting receptor potential (and, therefore, the action potential frequency in the relevant sensory nerve) tends to decline as time passes (Fig. 126). This is referred to as adaptation, since the receptor has adapted itself to the new stimulus level. Receptors like the Pacinian corpuscle, a pressure receptor in the skin, adapt very rapidly and so are more sensitive to changes in stimulus strength than to a constant stimulus. Such rapidly adapting receptors are said to be phasic. Tonic receptors, by comparison, adapt very slowly and so continue to generate sensory action potentials during sustained stimuli. Some of the proprioceptors in the musculoskeletal system behave in this way, providing continuous information about joint position.
7.4 Specific sensory systems
At the end of this section you should be able to:
Having considered the general properties of sensory systems, we can look at some specific details of individual modalities of sensation. In each case, we shall consider the receptors involved, a simple outline of the sensory pathways by which the information is relayed to the CNS and what is known about the processing of that information within the brain itself. Some of the more common sensory abnormalities will also be considered.
The somatosensory system
Somatosensory pathways
Information is relayed from the relevant peripheral receptors via unipolar sensory neurones which have their cell bodies in the dorsal root ganglia. The information enters the spinal cord through the dorsal nerve roots and is then transferred up to the brain (Fig. 127). Some sensory pathways (touch, pressure, temperature, pain) cross to the opposite (contralateral) side of the cord before ascending in the spinothalamic tracts. Others (proprioception, touch) travel up the dorsal columns on the same (ipsilateral) side of the cord before crossing the midline in the region of the medulla oblongata. Ultimately, therefore, all the sensory information from the right side of the body is routed through the left thalamus up to the left cerebral cortex. This explains why damage to the sensory pathways in the right cerebral hemisphere leads to sensory loss on the left side of the body. All sensory pathways also send inputs to the reticular-activating system in the brainstem area. This region is involved in controlling the level of consciousness, especially patterns of wakefulness and sleep. Increased sensory input increases awareness, making sleep less likely.
Somatosensory cortex
Although we may become aware of some cutaneous sensations (particularly pain) at the level of the thalamus, conscious awareness and localization of sensory stimuli is mainly a function of the somatosensory cortex in the postcentral gyrus of the parietal lobe (Fig. 128). Different parts of the body are represented by distinct regions within the sensory cortex. The site of origin of any stimulus is thus coded for in the brain by the anatomical position of the activated cortical neurones, an example of somatotopic representation. As a result, one can plot out a cortical map of the body, called the somatosensory homunculus. In this, the area of cortex devoted to a given region reflects touch sensitivity rather than anatomical bulk, e.g., the cortex representing the skin over the fingertips is more extensive than that representing the skin of the leg or back. This can be illustrated by drawing the body with each part scaled in proportion to the area of sensory cortex devoted to it—the so-called sensory homunculus (Fig. 129).
Somatosensory defects
Somatosensory damage within the brain is frequently caused by a stroke involving the internal capsule, a region carrying ascending tracts from the thalamus to the sensory cortex. This always produces sensory loss involving the opposite side of the body. There are usually associated motor defects, since sensory and motor axons lie in close proximity within the inner capsule (Section 7.5).
Pain
Types of pain
Somatic pain can be subdivided into:
Visceral pain has many causes including ischaemia and inflammation, as well as excess stretch or contraction of smooth muscle in hollow organs. It is often very hard to localize the source of visceral pain within the body, since we do not appear to have a neural map of our internal organs equivalent to the somatic map in the somatosensory cortex. In fact, visceral pain may appear to be coming from a part of the body anatomically distant from the true source, a phenomenon called referred pain. Ischaemic pain from the heart (angina), for example, may be experienced both as chest pain and as pain in the left arm. This seems to occur because sensory nerves from the heart enter the spinal cord at the same level as those from the arm. Similarly, irritation of the diaphragm produces pain referred to the shoulder tip since the sensory nerves from both diaphragm and shoulder enter the spinal cord in the cervical region. These are examples of the dermatome rule, i.e., pain from visceral afferents is often referred to the somatic dermatome whose sensory nerves enter the cord at the same nerve root level. Such considerations are important when seeking to interpret a patient’s description of their pain in terms of a possible cause.
Pain receptors or nociceptors
Pain seems to be detected by specific receptors, called nociceptors, and is not simply the result of overstimulation of other types of receptor. Structurally, nociceptors are bare nerve endings and are distributed more densely within the skin than are other sensory receptors. Some are sensitive to specific damaging stimuli, e.g., mechanical or thermal nociceptors, but others respond to a range of different noxious stimuli (multimodal pain receptors). Many are chemoreceptors sensitive to substances released within damaged tissues, regardless of the cause of that damage. These chemicals include normal cell constituents, such as K+ and ATP, which may be released following cell destruction, as well as inflammatory mediators like 5-hydroxytryptamine and bradykinin (Section 2.5).
Central pathways in pain perception
Incoming afferents from nociceptors synapse within the dorsal horn of the spinal cord. There are a number of spinal cord reflexes which may be triggered directly at this level, e.g., withdrawal from the painful stimulus (Section 7.5), but there is no conscious awareness of pain at the spinal cord level. Axons cross the midline and ascend the cord in the anterolateral spinothalamic tract (Fig. 127). This makes important collateral connections with the reticular formation in the brainstem, which activates the autonomic nervous system and increases general cortical awareness (painful stimuli increase arousal). The thalamus relays the pain signals on to the cortex. The parietal cortex is important in localizing pain while associated frontal lobe activity contributes to the accompanying distress.
Regulation of pain by endogenous opiates: pain gate
The transmission of signals from neurone to neurone in the pain pathways can be inhibited by the activity of other, pain-regulating pathways. A group of peptides known as endogenous opioids or opiates, which include the endorphins, enkephalins and dynorphins, play an important role in this activity. They are naturally produced within the body (endogenous) and bind to the same cell receptors as opiate analgesic drugs like morphine. Conditions associated with increased endogenous opiate production, including exercise and certain forms of emotional stress, are also known to have analgesic influences. One proposed mechanism for this is release of enkephalin from a descending, inhibitory pathway, which blocks the transmission of the pain signal within the spinal cord. This may rely on presynaptic inhibition in which the enkephalin reduces the release of excitatory transmitter (possibly substance P) from the incoming pain fibre. It is sometimes said that there is a pain gate in the spinal cord, controlling the onward transmission of pain signals. In this model, the descending enkephalin pathways act to close the gate, reducing pain perception. Inputs from other peripheral sensory nerves may also inhibit the transmission of pain within the CNS, although not necessarily at the spinal cord level. This may explain why rubbing the site of an injury brings some relief; the increased sensory input inhibits the central transmission of pain signals from that region.
Taste
Taste is dependent on chemoreceptors in taste buds within the mouth. These are particularly densely distributed along the sides of the papillae which cover the upper, or dorsal, surface of the tongue. The receptor consists of 40–50 chemosensitive cells which are exposed to the chemicals in their vicinity through an opening, or pore, in the upper surface of the taste bud (Fig. 130A). Microvilli, or gustatory hairs, project into this pore. Binding of a chemical to receptors on the microvilli causes release of neurotransmitter from the cell. This activates sensory nerves which connect the taste buds to the ipsilateral cortex (postcentral gyrus) via the thalamus, as well as sending relays to the brainstem and hypothalamus, regions involved in the control of feeding.
Taste is usually described in terms of one of four basic tastes, sweet, salt, sour (or acid) and bitter, and different regions of the tongue show varying sensitivities to each (Fig. 130B). These elementary tastes seem to reflect different overall patterns of taste bud stimulation rather than being caused by taste buds sensitive to only one type of chemical. Mammals are particularly sensitive to bitter tastes, which are often generated by chemicals in poisonous plants, presumably providing protection against their accidental ingestion.
Smell or olfaction
This depends on chemoreceptors in a small area of olfactory mucosa in the upper and posterior part of the nasal lining. The receptors are specialized neurones with microvilli projecting from their mucosal surface. Mucus-secreting cells support the receptor cells and molecules can only be detected once dissolved in the mucus layer. Axons emerging from the basal surface of the receptor neurones from the olfactory nerve, which passes through the bony cribriform plate and enters the olfactory bulb. This then connects directly with the olfactory cortex, which is part of the limbic system. The autonomic and behavioural controls exerted through hypothalamic and limbic mechanisms help explain the important emotional associations, and nervous and endocrine responses, which the sense of smell may evoke (Section 7.7).
The auditory system
Auditory thresholds and audiometry
The sensitivity of hearing varies with the frequency of sound, being maximal around 2000–3000 Hz (the upper frequency range in speech) and falling off above and below this value (Fig. 131A). Clinical audiograms do not always make this point clear, however, since the threshold pressure is often plotted in terms of hearing loss relative to the expected threshold at any given frequency, giving a normal value of zero for all frequencies (Fig. 131B).
Structure of the ear
The ear is divided into the outer, middle and inner ears (Fig. 132). The first two structures conduct sound to the cochlear portion of the inner ear where it is detected by specialized receptors. The vestibular apparatus, which is important for balance control, is also located within the inner ear and will be considered in more detail below.
Cochlear structure and function
The cochlea consists of a coiled tube which forms part of the bony labyrinth. In cross-section, the cochlea is seen to be divided into three chambers by two membranes. Reissner’s membrane (or the vestibular membrane) separates the scala vestibuli from the scala media (or cochlear duct), which is, in turn, divided from the scala tympani by the basilar membrane (Fig. 133). The scala vestibuli and scala tympani are filled with perilymph (similar in composition to cerebrospinal fluid) and are in continuity with each other via an opening at the very end of the basilar membrane known as the helicotrema. The scala media contains a K+-rich fluid known as endolymph and forms part of the membranous labyrinth in continuity with the vestibular apparatus (see Fig. 134).
Vibrations in the stapes are communicated to the fluid of the scala vestibuli via the oval window. Very-low-frequency movements simply displace fluid from the scala vestibuli to the scala tympani through the helicotrema and are not detected as sound. Higher frequencies, however, set the basilar membrane in motion and this movement is detected by hair cells in the organ of Corti, which generate an auditory signal. Basilar membrane vibration is possible because movement of the membranous round window, which opens back onto the middle ear, accommodates the displacement of fluid in the scala tympani.
Organ of Corti
The auditory receptor is the organ of Corti which lies within the scala media (Fig. 133). It sits on the basilar membrane and contains a number of hair cells, each projecting a series of stereocilia (the ‘hairs’ in question) from its upper pole. The ends of these cilia are embedded in the overlying tectorial membrane so that sound-induced vibration of the basilar membrane produces shearing movements between the cilia and the body of the hair cells. It is this distortion which sets up the receptor potentials in these specialized mechanoreceptors. Hair cells synapse with the neurones of the cochlear nerve, releasing excitatory transmitter when activated. The sensory nerve fibres carry auditory information centrally to the brain.
Pitch determination
Tonotopic coding: At higher sound frequencies, the frequency is coded for by the site along the basilar membrane at which hair cells are maximally stimulated, i.e., coding by place, rather than the pattern of action potentials in the auditory axons. Mechanical properties, such as the width and thickness of the basilar membrane, vary along its length. As a result, its resonant frequency also changes, being high (20 000 Hz) close to the oval window, and low (100 Hz) near the helicotrema (Fig. 134). Sound waves generate the largest amplitude of vibration at the point along the basilar membrane where the resonant frequency matches the frequency of the incident sound, and hair cell stimulation will be maximal at that point. Thus, the position of an activated hair cell along the length of the basilar membrane can be used to code for the frequency of a sound. Activity from receptors close to the base of the cochlea (the oval window end) is interpreted as being caused by a high-pitched sound.
Central auditory pathways
The cochlear nerves form part of the vestibulocochlear nerves (VIIIth cranial nerve, sometimes referred to as the auditory nerve) and travel to the cochlear nuclei in the medulla oblongata. From here the auditory pathways ascend to the medial geniculate nuclei in both the contralateral and the ipsilateral thalamus, sending collaterals to the reticular activating system en route. The thalamus, in turn, connects to the auditory cortex in the temporal lobe (Fig. 128).
Box 27 Clinical note: Deafness
Sensorineural deafness can be caused by damage to the cochlear hair cells following repeated exposure to loud noise. This usually produces more marked hearing loss at higher frequencies and may appear many years after sound exposure has ceased. A similar pattern of high-frequency deafness is also seen in many elderly subjects, a condition known as presbycousis (see Fig. 131B). Other causes of sensorineural defects include intrauterine infections such as rubella, leading to congenital deafness, and acquired deafness secondary to meningitis. Childhood deafness is a particularly severe handicap since it interferes with the acquisition of spoken language.
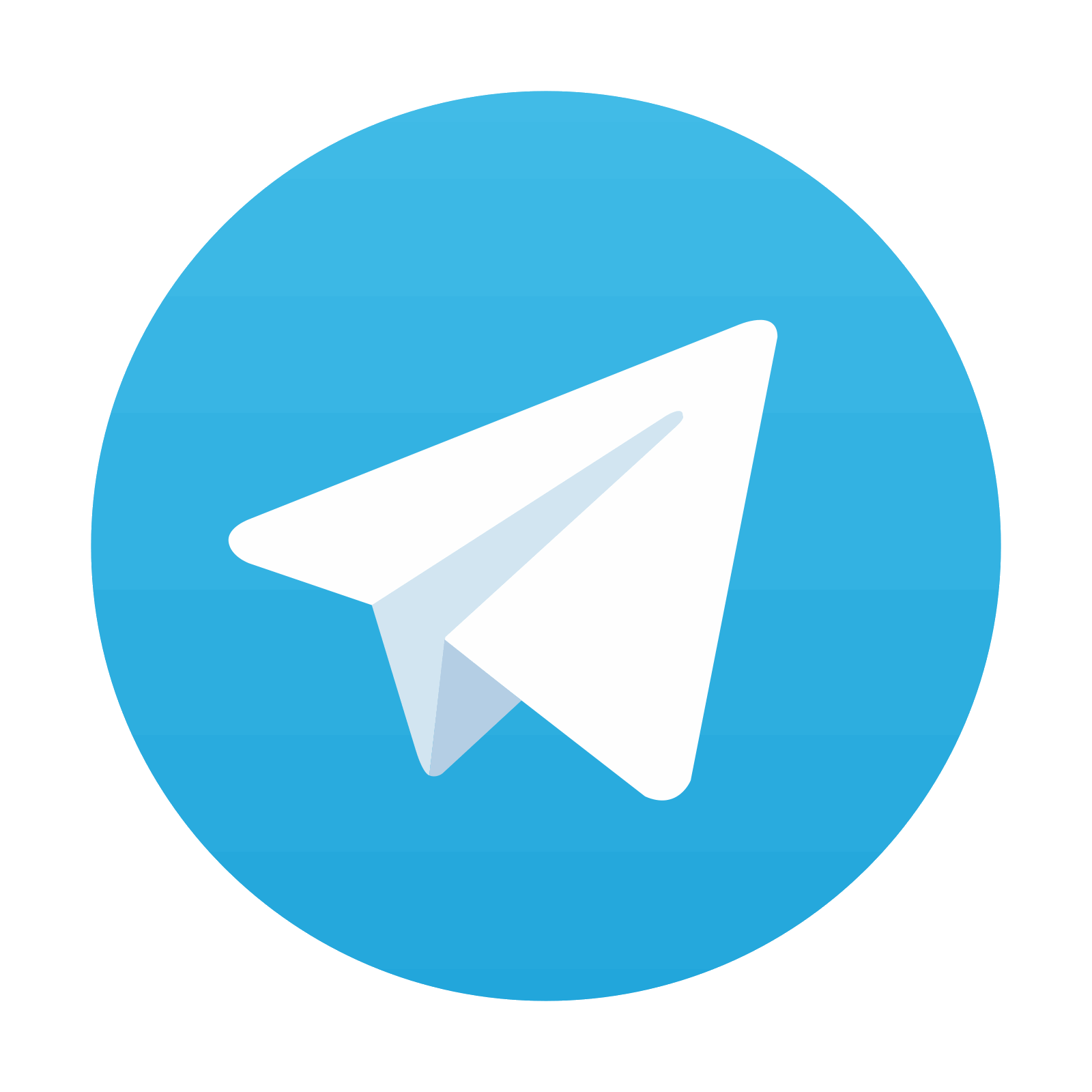
Stay updated, free articles. Join our Telegram channel
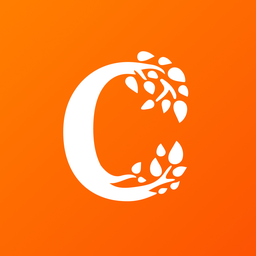
Full access? Get Clinical Tree
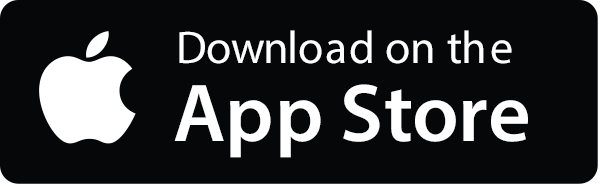
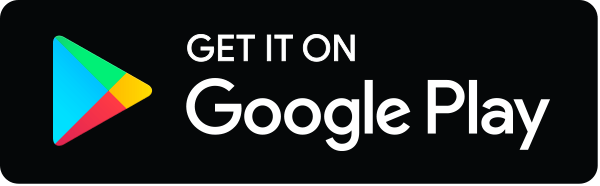