Ethanol (Alcohol)
History
Ethanol is surely the oldest known substance of abuse. The details of the original discovery of fermented beverages have been lost to time because of the unavailability of the written word. Thus no one really knows exactly when humans started drinking fermented beverages. Animals such as birds, insects, and elephants have shown signs of drunkenness by purposefully eating ripened fruit in which yeast produced ethanol. It is known that intentionally fermented beverages existed as early as 10,000 BCE. Aristotle, the founding father of the scientific method, showed in the 3rd century BCE that boiled wine lost its intoxicating character, but he never took the next step of condensing the ethanol. The distillation of ethanol from wine was discovered by a Muslim chemist in the 8th century. There are a myriad of accounts of the incorporation of alcoholic beverages into the daily life of every society. As with all substances of abuse, ethanol was probably not used abusively at first. For example, early versions of beer were used as food. Alcoholic beverages such as beer and wine were used in ritualistic religious exercises, and evidence of the use of beer and wine as medicinal remedies exist from at least 2000 BCE.
A recently published book describes in detail the cultural history of alcohol. The use of alcoholic beverages in most societies is certainly evident. It has produced social and health benefits as well as significant detrimental forensic and health problems. For example, light to moderate alcohol intake may be associated with lower risk of cardiovascular mortality in hypertensive men. On the other hand, excessive ethanol consumption is closely associated with abnormal elevation of blood pressure in normotensive and hypertensive individuals. Despite its social and possible health benefits, excessive ethanol consumption is a major medical health problem. In 1995, it was reported that about 10 million Americans were considered to be alcoholic.
Chemical Properties
Ethanol is a relatively simple molecule (C 2 H 6 O) with a molecular weight of 46 and density of 0.789 g/cm 3 . At ambient temperatures, it is a clear, colorless liquid that is highly volatile, flammable, and miscible with water and many other organic solvents. Its lipid-to-water partition coefficient is 0.096, so the distribution of ethanol favors an aqueous versus a lipid phase. In humans, this solubility ratio explains the distribution of ethanol in total body water.
Ethanol concentration is expressed in a variety of ways depending on the area of application. For example, a blood alcohol concentration of 0.1% (w/v) is the upper limit of the legal range while driving a car in many states. This concentration can also be expressed as 100 mg%, 100 mg/dL, 1 g/L, and 21.7 mM. The concentration of ethanol in commercially available forms of consumable, distilled alcoholic beverages is expressed in terms of proof, which is a number that is approximately double the percentage of ethanol (200 proof = 100% ethanol). Of interest, the term “proof” was used in the 19th century by English sailors who developed a test for the minimal concentration of ethanol in rum. If the sailor could successfully ignite gun powder soaked in the rum, it was proof that the rum was acceptably potent and had not been diluted with water. At least 50% (v/v) of ethanol is necessary to ignite gun powder. This simple test was important because the sailors were given rum as part of their pay.
Pharmacokinetics
Routes of Administration
Alcohol is one of the drugs of abuse that can be legally purchased, with age as the only restriction. Humans self-administer ethanol by the oral route exclusively. There are many forms of commercially available alcoholic beverages with widely varying percentages by volume, including beer (∼5%), malt liquor (∼7%), wine (∼12%), sherry or port (∼17%), cordials or liqueur (∼24%), brandy (∼40%), and distilled spirits (∼40%–50%).
Absorption and Distribution
After oral administration, ethanol is rapidly absorbed into the blood principally from the small intestine, but also from the stomach and colon. It is known that food, ethanol concentration, and liquid volume affect the gastric emptying rate and gastric absorption of ethanol, but once ethanol reaches the small intestine, its absorption is rapid and complete. For example, when a nonintoxicating dose of ethanol (∼0.5 g/kg) is ingested over a relatively short period of time (∼30 minutes) on an empty stomach, ethanol reaches its maximal concentration in the blood within 15–30 minutes. In fact, intravenous administration of ethanol combined with quantification of either breath or blood ethanol concentration has been used to estimate total body water.
Metabolism
The metabolism of ethanol occurs mainly in the liver by oxidation of ethanol to acetaldehyde by alcohol dehydrogenase and conversion of nicotinamide adenine dinucleotide to nicotinamide adenine dinucleotide hydrate. A secondary pathway of oxidative ethanol metabolism occurs in liver microsomal tissue in the smooth endoplasmic reticulum. Chronic consumption of ethanol increases the capacity of the liver microsomal ethanol oxidizing system, with an increase in several cytochrome P450s (CYPs), especially a nicotinamide adenine dinucleotide phosphate-requiring enzyme (CYP2E1). This system provides a higher rate of ethanol oxidation and is important to the development of tolerance to alcohol. Both of these mechanisms produce acetaldehyde, which is then converted to acetate by the action of aldehyde dehydrogenase.
Elimination/Excretion
The disappearance of ethanol from the blood or breath was originally thought to be a linear, zero order function, that is, independent of the blood ethanol concentration and probably due to saturation of alcohol dehydrogenase. However, it has been shown more recently that the elimination profile is more accurately described by Michaelis-Menten kinetics. Nevertheless, at higher doses of ethanol, there is an apparent linear phase (15 mg%/hour) that starts at the completion of the absorption-distribution phases and extends to a blood ethanol concentration of about 20 mg%. Below this concentration, the elimination becomes curvilinear. The elimination half-life of ethanol is about 2–4 hours. Between 90% and 95% of ingested ethanol is converted to acetaldehyde and acetate and then eliminated in the urine. About 3%–5% of a dose of ethanol is eliminated unchanged in the urine, breath, or through the skin. Less than 2% of ethanol is metabolized nonoxidatively to ethyl glucuronide, ethyl sulfate, phosphatidyl ethanol, and fatty acid methyl esters. Of interest, these direct metabolites of ethanol are measurable as markers for ethanol consumption.
Pharmacodynamics
The small size and simplicity of ethanol’s structure ( Fig. 17.1 ) is in contrast to its significant and complicated pharmacodynamic effects. When compared with most other drugs, very high concentrations of ethanol, in the millimolar range, are required to produce biological effects. Because of its simple chemical structure and the concentrations required for physiological effects, ethanol binding sites with high affinity (μM or nM range) most likely do not exist. It has been proposed that an ethanol binding site exists on γ-aminobutyric acid A (GABA A ) receptors that contain a combination of α4 (or α6), β3, and δ subunits, making them sensitive to concentrations of ethanol as low as 3 mM. Furthermore, relatively recent reports indicate that a certain domain of the enzyme adenylyl cyclase is responsible for sensitivity to ethanol. Amino acid mutations of target proteins designed to modify ethanol’s effects have suggested that specific groups of amino acid residues in transmembrane regions of proteins may be binding sites for ethanol. The results of these relatively recent studies suggest that ethanol situates itself into tiny pockets in protein structures to produce significant changes in biochemical, physiological, and behavioral functions (see Fig. 17.1 )

Neuropharmacological Effects
The neuropharmacological effects of ethanol have been identified and measured at biological levels from the molecular to the behavioral.
At the cellular/molecular level, it has been shown that acute, physiologically relevant concentrations of ethanol (5–50 mM) affect the function of numerous different receptor types. Ethanol inhibits N -methyl- d -aspartate (NMDA) receptors and l -type Ca 2+ channels and enhances the function of GABA A receptors, glycine receptors, serotonin-3 receptors, and neuronal nicotinic acetylcholine receptors.
Effects of ethanol on the aforementioned receptor systems (e.g., a neurotransmitter receptor or ion channel) can often be demonstrated using electrophysiological techniques, thereby showing effects on neurons or neuronal systems. If ethanol affects the rate of neuronal firing, membrane potential, or other cellular parameters, the effects of ethanol on receptor systems and perhaps specific brain areas can sometimes be linked to certain behaviors. Electrophysiological studies have shown that ethanol affects the firing rate of neurons in several brain areas, including the cerebellum, inferior olivary nucleus, locus coeruleus, ventral tegmental area, substantia nigra, hippocampus, and septal area. Furthermore, these effects of ethanol on single cells are thought to modify neurotransmission in the mammalian central nervous system, especially in the hippocampus, locus coeruleus, cerebellum, spinal cord, and cortex via pre- and postsynaptic effects.
From a behavioral perspective, acute low doses of ethanol are anxiolytic and can enhance mood and produce euphoric feelings in humans. In fact, the use of ethanol as a disinhibitor in social settings is well known and widespread throughout recorded history. For individuals who have the ability to limit ethanol intake, there appear to be social and health benefits. On the other hand, higher acute doses cause intoxication, sedation, sleep, and hangover, and very high acute doses can induce coma and possibly death as a result of respiratory depression. Studies with laboratory rodents showed effects of ethanol to produce hypothermia, analgesia, motor activation, and, at higher doses, motor incoordination. Chronic, heavy alcohol consumption produces a myriad of problems as discussed later in this chapter.
The behavioral consequences of acutely administered, lower doses (<30 mM) of ethanol may be linked to certain receptor systems. For example, intoxication, sedation, and motor incoordination are probably mediated via certain subunit configurations of the GABA A receptor. In addition, there is thought to be differential sensitivity among presynaptic, postsynaptic, and extrasynaptic GABA A receptors, with the extrasynaptic types being the most sensitive. Ethanol-induced hypothermia and analgesia are not thought to be mediated by GABA A receptors, but by inwardly rectifying G protein–gated K + channels. The concentration of ethanol required to affect other receptors is very high (>50 mM), so the involvement of these receptors at physiological concentrations is not clear.
A discussion of the neuropharmacology of ethanol would not be complete without comments about acetaldehyde. Acetaldehyde is the first metabolic product of ethanol in vivo and is produced by the action of alcohol dehydrogenase. Acetaldehyde may be responsible for some of the effects of ethanol, probably mostly the unpleasant and chronic side effects. The mechanism whereby these effects of acetaldehyde occur may be due to aldehyde-amine condensation products of amino acid side chains on proteins. Humans who carry a certain inactive form of mitochondrial aldehyde dehydrogenase do not metabolize acetaldehyde and have an uncomfortable facial flushing reaction after drinking alcohol. In addition, the hangover that occurs after a bout of heavy drinking is probably caused by high concentrations of acetaldehyde.
Toxicology
Whether ethanol produces an acute, satisfying, possibly beneficial effect versus an acute or chronic, unhealthy toxic effect is related to the concentration of ethanol, the dose of ethanol, and the length of exposure to ethanol. From a medical perspective, ethanol consumption of less than two standard drinks per day (≤ ∼30 g) by humans appears to decrease mortality and the mechanism is probably related to a reduction of coronary heart disease. Thus low or moderate levels of ethanol consumption are thought to be healthful. On the other hand, chronic heavy ethanol consumption of five standard drinks per day (∼75 g) or more for men and four standard drinks per day (∼60 g) or more for women, or frequent binging, can cause severe, detrimental health problems. These health problems result from direct toxic effects of ethanol on the liver, heart, brain, kidneys, and stomach. Indirectly, the replacement of calories from food by calories from ethanol (malnutrition) can cause additional negative effects on these organ systems. These serious effects of ethanol abuse can eventually result in alcoholism, a pathology that includes very serious medical and behavioral difficulties.
Cocaine
History
Cocaine is a derivative of the coca plant Erythroxylon coca , which is native to the mountains of South America. Traditionally, South American natives have chewed coca leaves as a stimulant to fend off fatigue, especially at relatively hypoxic elevations of the Andes Mountains. In 1574, the Spanish physician Nicolas Bautista Alfaro (1493–1588) published a description of the plant, its use, and its effects. In 1862, the German chemist Albert Neiman (Gottingen, Germany, 1834–1861) isolated the active component of the coca plant and called it cocaine. He was also the first to report the local anesthetic properties of cocaine, noting its numbing effects on his own tongue. In 1880, pharmacologist Basil Von Anrep of the University of Leipzig proposed use of cocaine as a surgical anesthetic in humans. Canadian surgeon William S. Halsted became the first physician to use cocaine for a nerve block during surgery and subsequently became the first known physician to develop a cocaine addiction. In 1884, psychiatrist Sigmund Freud published a cocaine monograph entitled Uber Coca in which he advocated for the use of cocaine to treat a variety of conditions including asthma, wasting diseases, and syphilis. Freud also eventually became addicted to cocaine. In the late 1800s, cocaine was added to a number of beverages including the “medicinal” wine Vin Mariani in France. In 1895, The Lancet published a report of six cocaine-associated deaths, underscoring the significant potential toxicity of this drug. Cocaine was an ingredient in the original version of John Pemberton’s Coca Cola; cocaine was removed from the popular soft drink in 1906. With passage of the Harrison Narcotics Tax Act in 1914, nonprescription use of cocaine was made illegal. In 1970 cocaine was classified as a Schedule II drug (Comprehensive Drug Abuse Prevention and Control Act).
Pharmacodynamics
Cocaine is a tropane (aminoester) alkaloid with a p K a of 8.6. Crack cocaine (free-base) is produced by combining cocaine HCl with an alkali. Crack cocaine is more heat-stable than is cocaine HCl and therefore can be smoked.
Cocaine interacts primarily with these central nervous system biogenic amine systems. Cocaine blocks the in vitro reuptake of norepinephrine, dopamine, and serotonin. Saturable, high-affinity Na + -independent and Na + -dependent [ 3 H]-cocaine binding has been associated with sites for serotonin and for dopamine reuptake, respectively, in the central nervous system. With acute or low-dose administration of cocaine, this reuptake inhibition leads to increased aminergic concentrations within aminergic synapses and increased binding both to pre- and postsynaptic aminergic receptors. Chronic cocaine administration is generally believed to result in aminergic depletion for the duration of drug exposure. However, unlike high doses of methamphetamine, chronic cocaine does not produce dopamine nerve terminal degeneration, at least in the prefrontal and frontal cortex or dorsal raphe.
Various addictive drugs such as cocaine that act as positive reinforcers increase synaptic dopaminergic concentrations in selected areas such as the nucleus accumbens. Homologous recombination targeting the dopamine transporter results in an absence of cocaine- or amphetamine-induced behavioral activation. The ventral tegmental area, nucleus accumbens, and caudate nucleus, areas rich in the neurotransmitter dopamine, are collectively considered the reward pathway. Cocaine antagonism of presynaptic dopamine transport results in elevated dopamine levels in mesolimbic synapses with resultant drug reinforcement. Maintenance of higher dopamine levels in reward pathway synapses leads to feelings of euphoria and a cocaine high. The increased availability of synaptic dopamine is thought to act on D1-like (D1 and D5) and D2-like (D2, D3, and D4) receptors in the mesocorticolimbic synapses. The effects of cocaine acting on the D1 receptor in the nucleus accumbens and ventral tegmental area are thought to be responsible, at least in part, for the reinforcing properties associated with long-term exposure to this drug.
Long-term cocaine use is associated with a selective decrease in D1 receptors in striatal reward areas both in rodents and in nonhuman primates. Dopamine depletion using the neurotoxin 6-hydroxydopamine infused into either the nucleus accumbens or the ventral tegmental area results in an attenuation of the reinforcing effects of cocaine self-administration. Similarly, dopamine D1 receptor knockout mice have demonstrated a lack of reinforcing effect of cocaine in contrast to wild-type controls. D1 and D2 receptors have opposing intracellular and behavioral effects and thus may differentially affect drug-reinforcing behaviors. David Self and colleagues demonstrated that cocaine self-administration behavior was mediated by dopamine D1 and not by dopamine D2 receptors and that this behavior progressively diminished in rats when cocaine was replaced with saline (extinction behavior).
Cocaine also blocks voltage-gated sodium channels and thus reversibly attenuates conduction of nerve impulses, which accounts for the local anesthetic properties of the drug. Depending on the relative distribution of the drug, a relatively selective depression of inhibitory neurons can produce cerebral excitation at lower concentrations of the drug, and this in turn can lead to generalized convulsions. At high concentrations, cocaine could produce more profound depression of brain function and ultimately coma, cardiorespiratory arrest, and death.
Pharmacokinetics
Distribution
Cocaine is rapidly absorbed across nasal, tracheal, and laryngeal epithelial membranes within minutes of its administration. Peak plasma concentrations of 120–474 ng/mL are reached within 30–60 minutes of intranasal administration and remain detectable up to 6 hours after administration. Cocaine may limit its own absorption due to its activity as a potent vasoconstrictor. Bioavailability of intranasal cocaine (i.e., area under the plasma concentration-time curve) is approximately 5 times less than that for equivalent intravenous dosing (0.19–2.0 mg/kg). The biological half-life for cocaine is 0.5–1.5 hours with a volume of distribution of 2.0 L/kg and a systemic clearance of 2.0 L/minutes.
Metabolism/Elimination
Cocaine metabolism is catalyzed by esterase activity, principally within plasma and liver. Liver esterase activity accounts for 30%–50% of the metabolism of cocaine to ecgonine methyl ester. Another 30%–40% of cocaine is nonenzymatically hydrolyzed into benzoylecgonine ( Fig. 17.2 ). The elimination half-lives of these major metabolites of cocaine are 6 and 4 hours, respectively. These metabolites can be detected in urine samples up to 60 hours after cocaine administration. A small amount (1%–5%) of cocaine is excreted unchanged in urine within 8 hours of administration. Individuals with liver dysfunction or malnutrition, or who are being treated with plasmapheresis, who are pregnant, or who have taken anticholinesterase medication (e.g., echothiophate eye drops, neostigmine) are relatively esterase-deficient, resulting in reduced capacity to degrade cocaine and thus elevated circulating levels of the drug with the potential for increased toxicity (see Fig. 17.2 ).

Cocaine metabolism demonstrates first-order kinetics over a wide range of doses. Within 4–5 hours, almost all of a dose of cocaine has been metabolized, with metabolites present in urine for 4–8 hours following intranasal dosing. . Measurable levels of the metabolite benzoylecgonine may be detected in urine as long up to 60 hours after a single dose of cocaine and for up to 3 weeks after heavy use of cocaine.
Less than 10% of cocaine is N -methylated into the active metabolite norcocaine. Liver N -methylation activity is increased by progesterone. N -methylation of cocaine into norcocaine is thus enhanced under conditions of elevated progesterone, for example, during pregnancy. This may account for the reported increased cocaine-associated cardiotoxicity during pregnancy.
Concurrent use of cocaine and alcohol results in the production of the active metabolite cocaethylene (ethylbenzoylecgonine) ( Fig. 17.3 ). Cocaethylene has a significantly longer elimination half-life than cocaine and may be more cardiotoxic than cocaine (see Fig. 17.3 ).

Toxicology
Cocaine-induced antagonism of nigrostriatal dopamine activity may result in extrapyramidal motor dysfunction including bradykinesia, akinesia, akathisia, catalepsy and dystonic reactions. In a study conducted between 1979 and 1990 by the medical examiner’s office for Dade County, Florida, excited delirium was associated with approximately one in every six cocaine-related deaths. These victims were described as having experienced an immediate onset of bizarre and sometimes violent behavior, including extreme paranoia, ending in cardiorespiratory collapse and death.
In addition, cocaine abuse is associated with both acute and long-term cardiotoxicity. Acutely, cocaine acts as a vasoconstrictor, reducing blood flow to myocardium with an increased risk for cardiac ischemia and infarction. Cocaine-inhibited reuptake of norepinephrine also leads to increased intracellular concentrations of calcium within cardiocytes, which through membrane depolarization can trigger sustained action potentials, extrasystolic contractions, and tachycardia. Long-term effects of cocaine on cardiac function can include a depression in contractility, in part related to the activity of cocaine as a local anesthetic. Cardiomyopathy associated with long-term cocaine abuse may be a result of oxidative stress to the myocardium. Systemic effects include peripheral vascular constriction with resultant ischemic compromise of various organs.
Long-term use of cocaine is also associated with elevated circulating levels of muscle enzymes such as creatine kinase, suggesting muscle degradation or rhabdomyolysis. Thirty-three percent of the participants in a study of cocaine-induced rhabdomyolysis experienced acute renal failure, severe liver dysfunction, and disseminated intravascular coagulation; six of them died.
Cocaine has been shown repeatedly to adversely affect reproductive function in female rats, male and female nonhuman primates, and male and female humans. In the rat, cocaine administration produces loss of estrous cyclicity, which at higher doses of cocaine may become permanent. Maternal cocaine use during gestation has also been associated with numerous adverse effects on the developing fetus, including growth retardation, dysmorphic features, seizures and strokes, and numerous postnatal behavioral abnormalities. Because such fetuses are typically exposed to various confounding variables, such as a lack of prenatal care, poor maternal nutrition, and polydrug exposures, the idea of a specific cocaine teratophilia (or “cocaine baby”) syndrome has been called into question.
Amphetamine and Amphetamine-Analogs
History
The Chinese plant ma hung ( Ephedra vulgaris ) has been used traditionally to treat asthma. In the 1920s the active ingredient in extracts of this plant was identified as ephedrine. In 1887, the Romanian chemist Lazar Edeleanu (1861–1941) first synthesized alpha-methylphenethylamine, now more commonly known as amphetamine ( a lpha- m ethyl ph en et hyl amine ). Originally named phenylisopropylamine, amphetamine was largely forgotten for the next four decades. In the late 1930s, amphetamine was prescribed for narcolepsy as well as hyperactivity syndromes. In 1932, the pharmaceutical company Smith, Kline & French marketed the racemic amphetamine ( dl -amphetamine) mixture Benzedrine as an over-the-counter medication for treating congestion by inhalation of the drug. Benzedrine was typically administered via inhalers. From its introduction in the 1930s until 1954, ephedrine was available without prescription (i.e., over-the-counter). By the 1940s and 1950s, reported abuse of these inhalers began to emerge. Benzedrine inhalers as well as other preparations of the drug could be used to produce a stimulant effect and were sometimes abused as “bennies.” With passage of the Comprehensive Drug Abuse Prevention and Control Act in 1970, methamphetamine and amphetamine became classified as Schedule II and III drugs, respectively. The following year, the classification of amphetamine was changed to Schedule II.
The term “amphetamines” also refers to a class of drugs derived from amphetamine, the substituted amphetamines. In recent years, the easily synthesized N -methylated form of amphetamine, methamphetamine (METH), has become readily available and one of the most commonly abused stimulants in the United States. But METH is not a recent addition to the list of amphetamine-related drugs. In 1885, the Japanese physician-chemist Nagayoshi Nagai (1844–1929) isolated ephedrine from the plant E. vulgaris and, in 1893, synthesized METH by reduction of ephedrine using red phosphorus and iodine. In 1929, he was the first to synthesize and elucidate the structure of ephedrine. In 1919, the Japanese chemist Akira Ogata (1887–1978) synthesized crystallized METH. In the 1980s, METH became increasingly more popular as a street drug. In 1996, the Methamphetamine Control Act was enacted, to regulate the key ingredients (e.g., ephedrine, pseudoephedrine, phenylpropanolamine), in manufacturing METH and increasing criminal penalties for possession, distribution, and manufacturing of the drug. The price of methamphetamine is largely determined by the availability of ephedrine, a key ingredient. Regulation of ephedrine, and its resulting unavailability, has increased its wholesale price. As a result, drug traffickers have switched to a less regulated and more economical substitute, pseudoephedrine. However, the Combat Methamphetamine Epidemic Act of 2005, a part of HR 3199, was enacted regulating over-the-counter sales of cold medicines containing pseudoephedrine. Methamphetamine can be smoked, snorted, or injected and has significantly longer-lasting stimulant effects than cocaine and is generally much less expensive to purchase than cocaine. Methamphetamine is considered highly addictive. Methamphetamine users typically develop tolerance to the drug that leads to higher and more frequent use.
Amphetamine is a homologue of phenethylamine and a weak base with a chemical structure very similar to that of dopamine and norepinephrine. Amphetamine is the parent compound for a class of similar psychoactive drugs including the N -methylated form of amphetamine, methamphetamine, and the methylenedioxy analog of methamphetamine, 3,4-methylenedioxy- N -methylamphetamine (MDMA; Ecstasy). Typically formulated as a racemic mixture ( d – and l -amphetamine), d -amphetamine is thought to act primarily on the dopaminergic systems, whereas l -amphetamine is comparatively norepinephrinergic (noradrenergic). Amphetamine and related drugs are lipid-soluble in nonionized form and as such are readily absorbed across the gastrointestinal tract lining as well as across the blood-brain barrier. Amphetamines can thus be taken effectively either by oral or parenteral routes of administration ( Fig. 17.4 ).

Pharmacologist David E. Nichols formulated the term “enactogen” to describe a class of synthetic drugs similar in structure to the stimulant MDMA (Ecstasy) but which exhibit hallucinogenic properties. The term “entactogen” is a combination of the roots “en” (Greek: within ), “tactus” (Latin: touch ), and “gen” (Greek: produce ). Enactogens are characterized by a substituted amphetamine core thus belonging to the phenethylamine class of psychoactive drugs. Enactogens include 3,4-methylenedioxyamphetamine (MDA or tenamfetamine), 4-methylenedioxy- N -ethylamphetamine, (MDEA), 3,4-methylenedioxy-alpha-ethyl- N -methylphenethylamine (MBDB; EDEN or Methyl-J), α-ethyltryptamine (etryptamine, α-ethyltryptamine, α-ET, or AET), and 4-bromo-2,5-dimethyphenethylamine (2C-B). MDMA is also often considered a member of the entactogen family.
4-Bromo-2,5-dimethyphenethylamine, or 2C-B, was first synthesized from 2,5-dimethoxybenzaldehyde by the Russian-American pharmacologist Alexander Shulgin in 1974. In the late 1970s, the German pharmaceutical company Drittewelle began manufacturing and marketing the drug as an aphrodisiac called Eros. Shortly after its introduction to clinical psychiatry, 2C-B made its way into the recreational drug scene. 2C-B remains popular within the rave subculture but is often confused with MDMA (Ecstasy). 2C-B and related entactogens are now classified as Schedule I drugs in the United States.
Based on the known mechanisms of action of MDMA, entactogens are thought to act by increasing synaptic levels of dopamine (DA), norepinephrine (NE), and serotonin (5-HT). However, there have been very few research studies to examine the pharmacology of entactogens in humans or experimental animal models.
Mechanism of Action
Amphetamines are CNS and sympathetic nervous system stimulants, acting on biogenic amine pathways. Physical effects include reduced appetite, hyperactivity, restlessness and insomnia, tachycardia, and increased blood pressure and constipation. Behavioral effects include anxiety and generalized excitability, a perception of increased energy, repetitive actions, increased alertness, emotional lability and, with higher or long-term dosing, occasional psychosis. These effects are similar to those of other stimulates such as cocaine. The amount of releasable as well as previously released DA at dopaminergic nerve terminals is closely regulated by two selective membrane-bound transporters. DA is moved via vesicular monoamine transporter-2 (VMAT-2) into synaptic vesicles for storage and eventual release. Without vesicular transport, DA remains within the cytoplasm where it is subject to degradation including oxidation and presynapse via the DA transporter (DAT). DAT inhibition or blockade results in increased levels of extracellular DA. Amphetamines induce presynaptic DA release, block DA reuptake, inhibit DA storage within presynaptic vesicles, and block enzyme-catalyzed DA metabolism. Shortly after amphetamine administration, reversal of the DAT results in nonvesicular DA efflux. In addition, the movement of DA into synaptic vesicles is blocked by amphetamine, resulting in increased DA release into the synapse and the potential for DA oxidation and damaging free radical formation within the presynapse. Although also acting through similar mechanisms on NE and, to a lesser extent, 5HT terminals, amphetamine’s reinforcing and behavioral-stimulant effects are associated with enhanced dopaminergic activity, primarily within the mesolimbic DA system. The targeted effects of amphetamine on dopaminergic activity in caudate nucleus, nucleus accumbens, and ventral striatum correlate well with stereotypic onset of euphoria associated with this drug. Amphetamine also acts to increase glutamate release in selected brain areas such as the nucleus accumbens, striatum, and prefrontal cortex. These areas are implicated in reward pathways.
In similar fashion to the DA transporter (DAT), amphetamine can also induce reverse the direction of serotonin movement via the serotonin transporter (SERT). Amphetamine interacts with the serotoninergic system in selected brain regions such as the mesocorticolimbic pathway. Similar to amphetamine, methamphetamine induces release of 5HT, DA, and NE as well as blockade of 5HT, DA, and NE transporters within the CNS, leading to increased synaptic activities of these biogenic amines.
Formation of free radicals including oxygen and nitrogen species (ROS and RNS, respectively) is particularly characteristic of methamphetamine administration. An amphetamine analog, methamphetamine, induces selective degeneration of DA neuron terminals without cell body loss. Methamphetamine-induced terminal degeneration may be mediated in part by excitatory amino acid (e.g., glutamate) activity. Methamphetamine also induces rapid and reversible decreases in the rate-limiting enzyme for serotonin (5-hydroxytrypamine, 5-HT) synthesis, leading to reduced levels of this biogenic amine within various CNS areas.
Amphetamine, MDMA, and other psychotropic agents may also interact with a relatively new class of receptors called the trace amine-associated receptors (TAARs). TAARs represent a class of G protein–coupled binding sites for endogenous trace amines, metabolic products of the better-known biogenic amines such as NE, DA, 5HT, and histamine. Trace amines are normally present in very low (nanomolar) concentrations and include tyramine, octopamine, tryptamine and β-phenylethylamine. Trace amines such as β-phenylethylamine may function to modulate biogenic amine synaptic activities in select brain areas related to affective states and thus in maintaining levels of excitement and alertness. Branchek and Blackburn have hypothesized a role for trace amines in substance abuse, depression, attention-deficit/hyperactivity disorder, eating disorders, schizophrenia, and other neuropsychiatric diseases.
Pharmacokinetics
Metabolism and Elimination
Metabolism of amphetamine is almost exclusively hepatic. In the liver, amphetamine can be hydroxylated (phenyl ring), deaminated, or conjugated. Methamphetamine is N -demethylated. In a study of human subjects given measured doses of amphetamine orally, 34% of the drug was excreted unchanged in urine. Metabolites of the drug included benzoic acid and parahydroxy-amphetamine. These metabolites were themselves further converted into hippuric acid and parahydroxyephedrine, respectively.
Toxicology
The toxic effects of amphetamine and related drugs can be dangerous and potentially fatal. Short-term effects of amphetamine include increased heart rate and blood pressure, decreased appetite, feelings of elation and self-assuredness, and reduced fatigue. Long-term, repetitive amphetamine use has been associated with insomnia and restlessness, significant weight loss, hallucinations, and paranoid psychosis. Amphetamine use can also include psychic dependence and tolerance as well as psychotic episodes in some individuals. Continuous high-dose amphetamine use has been associated with a state of paranoid (amphetamine) psychosis closely resembling the symptoms of paranoid schizophrenia. Symptoms include hyperactivity, anxiety, paranoid delusions, and auditory-tactile hallucinations in a setting of clear consciousness with little if any disorientation.
Use of amphetamines and related stimulants is particularly dangerous in patients with a history of heart disease or underlying hypertension as well as patients with glaucoma. Amphetamines and stimulants should also be avoided by anorexic individuals because of the appetite- suppressing properties of these drugs. In addition, amphetamines can cause life-threatening hypertensive crisis and possibly neurotoxic reactions when taken with monoamine oxidase (MAO) inhibitors for clinical depression. Amphetamine-associated death is considered to be a direct consequence of excessive sympathomimetic activity. Amphetamine toxicity includes hypertension, hyperpyrexia, delirium, convulsions, and severe tachycardia leading to cardiovascular collapse. Amphetamine also increases sympathetic tone, regulating smooth muscle contraction and thus can affect adversely the functions of the gastrointestinal tract, uterus, urinary bladder, and other organs dependent on smooth muscle activity.
MDMA has been associated with serotoninergic depletion and neuronal degeneration in rodent and nonhuman primate models. These neurotoxic effects were more pronounced in nonhuman primates than in rodents. Within 3 weeks of MDMA administration, profound serotoninergic neurodegeneration was seen in nonhuman primates in most brain areas. Some CNS areas showed evidence of partial recovery, that is, hippocampus, caudate nucleus, and frontal cortex. However, the partial recoveries appeared to be short-term, with a return to the dramatic patterns of serotoninergic losses seen 2 weeks after drug exposure.
Colado and colleagues reported that MDMA administration to pregnant rats does not produce damage to 5-HT nerve terminals in the brains of the fetuses, in contrast to the serotoninergic neurodegeneration seen in the CNS of the mothers. They hypothesized that this contrast in maternal versus fetal effects may be due to MDMA converted into free radical–associated metabolites in the adult brain but not in the immature brain. Alternatively, the developing CNS may have more effective or more active free radical scavenging mechanisms than the mature adult CNS.
Therapeutic administration of amphetamines is usually by the oral route. When used recreationally/illicitly, amphetamines are taken orally, snorted, smoked, or by intravenous injection. Methamphetamine’s methyl group is lipid soluble and easily transported across the blood-brain barrier as well as relatively resistant to enzymatic degradation catalyzed by MAO activity. After an oral administration (4 x 10 mg), methamphetamine is initially detected in plasma samples within 15 minutes to 2 hours. Maximal plasma concentrations (14.5–33.8 μg/L) are achieved within 2–12 hours and the drug remains measurable for 36–72 hours after administration. Methamphetamine has an elimination half-life of 9–15 hours primarily via urinary excretion. Methamphetamine elimination half-life varies with differences in urinary pH. One of the metabolites of methamphetamine is amphetamine.
Amphetamine and amphetamine-like derivatives are potent CNS stimulants affecting regulatory centers for heart rate, body temperature, blood pressure, appetite, attention, mood, and responses associated with alertness or alarm responses. Physiological and psychological responses to amphetamines closely resemble the sympathetic nervous system–induced fight-or-flight responses, including increased heart rate and blood pressure, vasoconstriction, bronchodilation, and hyperglycemia. Users report increased ability to focus on tasks, an overall increase in mental alertness, avoidance of fatigue, and decrease in appetite.
Drug tolerance develops rapidly in amphetamine abuse. Tolerance to the drug’s effects results in increasing amounts of the drug needed to obtain similar rewarding effects. However, chronic amphetamine use can produce so-called reverse tolerance, or sensitization to some of the psychological effects of the drug. Amphetamine users will often take more of the drug during withdrawal periods and may use other drugs such as benzodiazepines, or less commonly, barbiturates to lessen the effects of withdrawal.
Methamphetamine administration is associated with both dopaminergic and serotoninergic neurodegeneration. The generation of free radicals as a byproduct of increased DA and 5HT metabolism is postulated to play a key role in this neurodegeneration. Blocking increases in METH-induced release of DA or 5HT reduce the neurodegeneration seen with administration of this drug. In addition, pretreating with multiple injections of escalating doses of methamphetamine produces tolerance to the long-term neurotoxic effects of methamphetamine on striatal DA neurons. Although not yet well defined, the mechanism for this tolerance may be related to aberrant monoamine transporter-2 (VMAT-2) and dopamine transporter function in these neurons.
Cathinone and Synthetic Cathinone Derivatives
History
The leaves of the Catha edulis (khat) plant, which is native to the Horn of Africa and Arabian Peninsula, have been chewed for thousands of years, purportedly for their stimulant-like effects. In 1930, Wolfes identified the primary active constituent of khat to be cathine [(+) pseudonorephedrine]; cathinone [β-keto-amphetamine] was later identified to be a more potent contributor to the stimulant effects of khat and for which cathine is a primary metabolite.
Recent estimates suggest that khat is used daily by approximately 20 million people 88 ; however, because its use is concentrated in the Arabian Peninsula and portions of Eastern Africa, it is not considered to a represent a major public health problem worldwide. Nevertheless, khat has received significant attention from international regulators, with its potential for abuse/dependence first discussed by the League of Nations in 1935, and again by the United Nations and World Health Organization at the Single Convention on Narcotic Drugs in 1961. However, it was not until the Convention on Psychoactive Substances in 1971 that cathinone was placed on the international list of Schedule I drugs. Subsequently, the US Drug Enforcement Administration (DEA) added cathinone to its list of Schedule I drugs in 1993.
Because of its use as a precursor to the chemical synthesis of ephedrine and norephedrine, cathinone was first synthesized in 1915 by Eberhard. With slight modifications to Eberhard’s synthesis, Adams and colleagues (Hyde et al., 1928) developed a method for synthesizing cathinone and several derivatives of cathinone that are still in use today. Driven in large part by chemical and pharmacological similarities among cathinone, ephedrine, and the amphetamines, numerous cathinone derivatives have been synthetized and patented by drug companies for their putative antidepressant and/or antiparkinsonian effects. For instance, methcathinone (α-methylamino-propiophenone) was first patented in Germany in 1936 (German Patent 639,126) as a chemical precursor for the synthesis of ephedrine (and ephedrine derivatives), later used in the Soviet Union for its antidepressant properties (1930s to 1940s), and subsequently patented by Parke Davis in 1957 (US Patent 2802865). Because of concern for abuse, methcathinone was listed as a Schedule I drug in 1994 by the International Convention on Psychotropic Substances, as well as the DEA.
Although recreational use of methcathinone in the 1970s provided early indications that synthetic cathinones possess high abuse potential, it was not until mephedrone (4-methylmethcathinone) and methylone (3,4-methylenedioxy- N -methylcathinone) emerged on the European club scene in around 2000 that these cathinone derivatives gained widespread popularity among drug abusers. Since that time, the recreational use of synthetic cathinones has increased dramatically around the world. They are widely available, marketed as “bath salts,” “research chemicals,” or “plant food,” and can be easily purchased on the Internet and in local shops as “safe” and “legal highs.” Indeed, anecdotal and survey data suggest that their effects are similar to those produced by cocaine and methamphetamine. Coincident with the increasing popularity of these agents as recreational drugs, poison control centers and emergency departments around the world have seen dramatic increases in the number of patients presenting with cardiovascular and/or neuropsychiatric toxicities as well as overdose fatalities related to the use of synthetic cannabinoids and/or cathinones. Because of the rapid increase in abuse and toxicity associated with the recreational use of these synthetic cathinone derivatives, in 2011 the DEA used its emergency scheduling authority to temporarily list three synthetic cathinones (mephedrone, methylone, and MDPV) as Schedule I. Although these regulations temporarily reduced the production/sale/use of these particular compounds, new derivatives with similar pharmacologic properties, and presumably high potential for abuse, quickly took their place in the market. In 2014, the list of synthetic cathinones placed under Schedule I was expanded to include 10 additional derivatives (4-MEC [4-methylethcathinone], 4-MePPP [4’-methyl-α-pyrrolidinopropiophenone], α-PVP [α-(pyrrolidin-1-yl)valerophenone], butylone [β-keto- N -methylbenzodioxolylbutanamine], pentedrone [α-methylamino-valerophenone], pentylone [β-keto-methylbenzodioxolylpentanamine], 4-FMC [flephedrone; 4-fluoromethcathinone], 3-FMC [3-fluoromethcathinone], naphyrone [naphthylpyrovalerone], and α-PBP [α-(pyrrolidinobuterophenone]), bringing the total number of synthetic cathinones listed as Schedule I drugs to 13 as of 2016.
Chemical Properties
Similar to amphetamine, cathinone is a chemical derivative of phenethylamine; however, in addition to the alkyl group on the alpha carbon, cathinone also contains a ketone group on the beta carbon (i.e., β-keto-amphetamine). This core cathinone structure (phenethylamine with an alkyl group on the alpha carbon and a ketone group on the beta carbon) contains four locations for chemical modification (see Fig. 17.1 ), and is conserved across the more than 200 chemical derivatives of cathinone that have been described. Despite similarities among the chemical structures of the synthetic cathinones, the cathinone superfamily has a relatively heterogeneous pharmacology, with two main subfamilies of compounds defined by their capacity to inhibit the uptake monoamines by their transporters (i.e., inhibitors), or to both inhibit the uptake and stimulate the release of monoamines (i.e., substrates) ( Fig. 17.5 ).

Pharmacokinetics
Cathinone and its derivatives are readily absorbed and have high blood-brain barrier permeability, with behavioral effects apparent within minutes after systemic administration in rodents. a Although the pharmacokinetic profiles of cathinone and several of the abused cathinone derivatives (e.g., MDPV, α-PVP, mephedrone, and methylone) have been examined, little is known about the pharmacokinetic properties of the majority of the synthetic cathinones. As with most amphetamine-like compounds, cathinone and its derivatives are metabolized primarily by liver enzymes. Although it is well known that cathinone is metabolized to pharmacologically active compounds (i.e., cathine and norephedrine), less is known about the pharmacologic activities of the metabolites of other cathinone derivatives. Of interest, whereas some of the synthetic cathinones (e.g., MDPV) exhibit linear pharmacokinetics, other cathinone derivatives exhibit nonlinear pharmacokinetics. For example, whereas methylone is metabolized primarily by CYP2D6, it is also known to function as a mechanism-based inactivator of CYP2D6 function, effectively inhibiting its own metabolism in a time- and dose-dependent manner.
a References .
Pharmacodynamics
Similar to other CNS and sympathetic nervous system stimulant drugs, cathinone and its synthetic derivatives increase extracellular levels of monoamines through their interactions with transporters for dopamine (DAT), norepinephrine (NET), and serotonin (SERT). As mentioned earlier in this chapter text, chemical diversity within the cathinone superfamily has resulted in subfamilies of compounds with slight, but important, differences in their primary mechanism of action. Similar to amphetamine, cathinone and some of its derivatives (e.g., substrates such as mephedrone, methylone, and 3-FMC) are known to both inhibit the uptake and stimulate the release of dopamine, norepinephrine, and serotonin, whereas other cathinone derivatives (e.g., inhibitors such as MDPV, α-PVP, naphyrone) are capable of inhibiting monoamine uptake without stimulating their release, a mechanism of action more similar to that of cocaine than amphetamine. In addition to differences in their effects at monoamine transporters (i.e., release and/or inhibition), a great deal of diversity exists among the synthetic cathinones with respect to the selectivity with which they act at dopamine, norepinephrine, and serotonin transporters, as well as other nontransporter sites. For example, among inhibitors, some compounds (e.g., naphyrone, pentylone) inhibit the uptake of dopamine, norepinephrine, and serotonin at comparable doses, whereas other compounds, including MDPV, and α-PVP, are potent inhibitors of dopamine and norepinephrine uptake with low affinity for the serotonin transporter. Similar differences are also apparent among substrates, with compounds such as cathinone being more potent at releasing dopamine than serotonin, whereas other cathinone derivatives, such as mephedrone, are equipotent at releasing dopamine and serotonin.
Although relatively little is known about the effects of synthetic cathinones at sites other than the monoamine transporters, affinity for serotonin (1A, 2A, and/or 2C) and/or adrenergic (α1, α2a) receptors has been reported for some cathinones. Similar binding profiles have been reported for other amphetamine-like compounds (e.g., amphetamine, methamphetamine, MDMA); however, it is worth noting that unlike many amphetamine-like compounds, beta-keto containing cathinones appear to be devoid of activity at TAARs.
To date, the majority of preclinical studies on the behavioral effects of cathinones have focused on characterizing their acute locomotor effects. b Although the discriminative stimulus and reinforcing effects of cathinones have been described in much less detail, preliminary evidence is generally consistent with human reports as well as early studies of behavioral effects of cathinone and methcathinone in laboratory animals. c For instance, studies in rats and mice suggest that the common bath salts constituents MDPV, mephedrone, methylone, α-PVP, α-PBP, methcathinone, pentedrone, pentylone, naphyrone, flephedrone, 3-FMC4-MEC, and 4-MePPP all produce cocaine- and/or methamphetamine-like discriminative stimulus effects, d and that MDPV, mephedrone, and methylone, function as reinforcers when delivered intravenously. e Although MDPV, mephedrone, methylone, and α-PVP are all capable of maintaining responding in standard self-administration assays, there is still much we do not know about the factors that contribute to the purported high abuse potential of bath salts, including whether their abuse-related effects are altered when administered in combination with other bath salt constituents.
b References .
c References .
d References .
e References .
Toxicology
Although national drug use surveys (e.g., Substance Abuse and Mental Health Services Administration [SAMSHA] National Survey on Drug Use and Health) have yet to be updated to include data on synthetic cathinone (i.e., bath salts) overdoses, the Drug Abuse Warning Network recorded over 20,000 emergency department visits involving bath salts in 2011 alone. 74 As with other stimulants, the most common symptoms associated with bath salts overdose are cardiovascular (e.g., tachycardia, hypertension, and chest pain) and/or neuropsychiatric complications (e.g., agitation, paranoia, and hallucination (see references 19, 199, and 237). Whereas acute myocardial infarction can seriously compromise the health of the user, case reports suggest that the paranoia and hallucinations produced by bath salts can precipitate self-destructive or violent behavior that can put users, their families, and medical staff at risk. (Stimulants such as cocaine, methamphetamine, and MDMA have well-characterized cardiovascular effects in rats and preliminary evidence suggests that mephedrone and MDPV produce similar, if not greater, increases in heart rate and mean arterial pressure. ) Given the overlap between the toxic effects of cathinones and other stimulants (e.g., cocaine, methamphetamine, MDMA), it is not surprising that current treatments for cathinone overdose parallel those used for cocaine, including the use of benzodiazepines to decrease cardiovascular effects and agitation. In some cases, haloperidol has been used to decrease the central stimulant effects of cathinones; however, as with cocaine, beta-blockers are contraindicated for the treatment of hypertension because they have been shown to result in further increases in blood pressure.
However, it should be noted that because bath salts preparations often contain multiple psychoactive compounds, with mixtures of two cathinones, and mixtures of a cathinone and caffeine being the most common, f it is difficult to say whether the toxicities observed in bath salts can be attributed to a single cathinone, or whether they result from interactions among the various bath salts constituents.
f References .
Opiates
History
Crude opium is a component of the opaque, milky-white sap obtained from the seedpods of the poppy plant ( Papaver somniferum ). This plant and its product opium were likely cultivated in the Mediterranean region as early as 5000 BCE by ancient Egyptians and Greeks and later by the ancient Romans. Opium was introduced into China around BC 800 and, with the arrival of European explorers to China, to Europe by the early 18th century. In 1680, a famous English physician named Thomas Syndenham introduced opium to the medical field. In the 17th century, many people in Europe were treated for a variety of health problems with opium. In 1729, opium smoking was made illegal in China and soon the importation of opium was banned. This ban upset the British who were in charge of trading this valuable product. Opium was still smuggled into China and is considered the underlying cause of the so-called Opium Wars (1839–1842 and 1856–1860) between the British and the Chinese. In the United States, opium was used to treat soldiers during the Civil War (1861–1865). During the late 1800s, doctors prescribed “tonics” containing opiates for many conditions. Typically, these medicines failed to list opiates as one of the ingredients.
Opium represents a complex of sugars, proteins, fats, water, meconic acid, plant wax, latex, gums, ammonia, sulfuric and lactic acids, and numerous alkaloids. Alkaloids present in opium include morphine (10%–15%), codeine (1%–3%), papaverine (1%–3%), and thebaine (1%–2%). Noscapine, narceine, and approximately 25 other alkaloids are also present, but have essentially little to no effect on the CNS and are not usually considered to be opiates. Thebaine is considered highly toxic and thus not used therapeutically ; it acts as a potent stimulant rather than as a depressant, at higher concentrations, causing strychnine-like convulsions. Thebaine can be used to produce the semisynthetic morphine analogues oxycodone, dihydromorphinone, hydrocodone, and etorphine.
In 1805 the German pharmacist Frederick Serturner isolated morphine from opium. He named this newfound compound after Morpheus, the Greek god of dreams. Morphine-related analogues include the diphenylpropylamines (e.g., methadone), the 4-phenylpiperidines (e.g., meperidine), the morphinans (e.g., levorphanol), and 6,7-benzomorphans (e.g., metazocine), each of which has in common a piperidine ring or a key component of that ring structure. Heroin was first synthesized in 1874 by the British chemist C. Adler Wright. Heroin is synthesized from morphine. Heroin is the 3, 6-diacetyl ester of morphine, that is, diacetylmorphine (see Fig. 17.5 ). Heroin became widely accepted within the medical community in the early 1900s. The high risk for addiction was not initially recognized by physicians at that time. With a better understanding of its abuse potential, heroin was later regulated with passage of the Harrison Narcotics Tax Act of 1914. The drug is now classified as a Schedule I substance with significant abuse potential but no accepted medical use.
The term “opiate” refers to morphine-like alkaloids (e.g., morphine itself, heroin, codeine, thebaine, and papaverine) derived from opium as well as a number of semisynthetic and synthetic opiates. Opioid refers to endogenous substances with morphine-like activity. Endogenous opioids include dynorphins, enkephalins, endorphins, endomorphins, and nociceptin/orphanin FQ. The term “endorphin” was first used in the mid-1970s to describe any endogenous morphine-like substance, now classified as endogenous opioidergic peptides. We now recognize several classes of endogenous opioidergic peptides including enkephalins, endorphins, dynorphins, and endomorphins. Opiates such as morphine and heroin bind to and activate the same receptors used by endogenous opioidergic peptides, namely mu (μ), kappa (κ) and delta (δ) receptors. Opioidergic receptors are expressed only in the CNS, gastrointestinal tract, and vas deferens. Most opioid receptors are linked to an inhibitory G protein (G i ). Thus endogenous opioidergic peptide receptor binding is associated with inhibition of adenylate/cAMP second-messenger systems and inhibition of the target neuron.
Endogenous opioidergic peptides are generally involved with homeostasis or regulation of basic physiological functions including respiration, endocrine functions, and nociception (pain). Endogenous opioidergic peptides may also play important roles in mood and affect. The greatest density of opioidergic receptors is found in the limbic system (emotions and affect) and in the dorsal horn of the spinal cord (nociception). Thus it should not be surprising that endogenous opioidergic peptides are thought to integrate euphoric and emotional components of pain relief ( Fig. 17.6 ).

According to the Department of Health and Human Services, the United States is currently in the midst of an opiate epidemic. Prescription opiates are now the most commonly prescribed class of drugs in the United States, and although they are effective and an important component of balanced pain management, the increased clinical use is associated with increased risks. For example, abuse of prescription opiates has increased dramatically in the last two decades, with more than 10 million people in the United States using prescription opiates nonmedically. As a direct result of increased abuse, there has been a dramatic escalation in overdose deaths. Nearly 500,000 people in the United States died from a drug overdose between 2000 and 2014, with more than 61% of those fatalities attributed to opiates. Moreover, the death rate from opiate overdose has grown every year since 2000, and the rate of fatal overdose increased 14% from 2013 to 2014. Prescription opiate abuse is also fueling an increase in heroin abuse, because heroin is often cheaper and more accessible than other opiates ; as a result, the death rate due to heroin overdose increased 26% from 2013 to 2014. In addition to declaring that opiate overdose fatalities have reached epidemic levels, the US Department of Health and Human Services now advocates for greater access to treatment for opiate abusers. In addition, the Centers for Disease Control and Prevention has issued new guidelines for prescribing opiates that includes 12 recommendations for clinicians who are treating patients with chronic pain.
Mechanism of Action
Opiate agonists and antagonists bind to stereospecific, saturable receptors in the brain and other tissues. CNS opioidergic receptors are widely but unevenly distributed. These receptors were originally classified according to their affinity for binding agonists: mu (μ) receptors preferentially bind to morphine, kappa (κ) receptors to ketocyclazocine, and delta (δ) receptors to deltorphin II. Morphine also binds to both kappa and delta receptors, although with less affinity than to mu receptors. In 2000, these receptors were reclassified as OP 1 (delta), OP 2 (kappa), and OP 3 (μ) by a subcommittee of the International Union of Basic and Clinical Pharmacology. Mu receptors are located widely throughout the CNS, especially in the limbic system (frontal and temporal cortex, amygdala, and hippocampus); thalamus; striatum; hypothalamus; and midbrain. Kappa receptors are located primarily in the spinal cord, periaqueductal gray area, and cerebral cortex. Delta receptors are located primarily in pontine nuclei, amygdala, olfactory bulbs, and deep cerebral cortex. Opiate receptors are G-protein-coupled and function as modulators, both positive and negative, of synaptic transmission. Most known opiates exhibit no ceiling effect for analgesia with the exception of codeine for which a ceiling effect is estimated as 7mg/kg. Mu receptor activation results in analgesia, euphoria, respiratory depression, miosis, decreased gastrointestinal motility, and physical dependence. Kappa-receptor stimulation also produces analgesia, miosis, respiratory depression, as well as dysphoria and some psychomimetic effects (i.e., disorientation and/or depersonalization). Delta receptor activation produces dysphoria, is hallucinogenic, and produces respiratory depression at high doses and cardiac stimulation. Opiates working primarily through mu receptors also suppress cough reflex. The antitussive effects of codeine are mediated through direct action on receptors in the cough center of the medulla.
Heroin is transported rapidly across the blood-brain barrier and metabolized into morphine and related compounds. It is generally assumed that heroin itself has only minor pharmacological effects. Most of the pharmacological effects associated with heroin are actually caused by morphine as well to some degree, the other two major metabolites 6-acetylmorphine (6-AM) and metabolites 6-acetylmorphine (M6G). M6G possesses analgesic properties, although M3G does not seem to have any agonistic effect either in vivo or in cell cultures.
Most opiates such as heroin and morphine produce a profound sense of euphoria in most users, although this effect diminishes with development of tolerance to the drug. Heroin and morphine share in common the ability to induce euphoria, relaxation, as well as, with time, drowsiness and sleepiness. Short-term studies among users suggested that heroin tolerance develops no more rapidly than tolerance to morphine. This is perhaps not surprising given the physicochemical properties of heroin and morphine and the metabolism of heroin metabolism to morphine.
Pharmacokinetics
Routes of Administration and Metabolism
Heroin represents the diacetyl derivative of morphine. The usual route of administration is intravenous, although other routes of administration include intramuscular, subcutaneous, rectal, and intranasal. After absorption, it is rapidly converted into either morphine or monoacetylmorphine, which is highly lipid soluble and thus easily crosses the blood-brain barrier with rapid induction of euphoria. When taken orally, heroin undergoes extensive first-pass metabolism via deacetylation into morphine in the liver. Thus heroin can be considered a prodrug. In contrast, intravenous injection of the drug essentially bypasses first-pass metabolism with rapid distribution across the blood-brain barrier due to the presence of acetyl groups, which makes the drug more lipid soluble than morphine. Within the brain, heroin is deacetylated into 3- and 6-monoacetylmorphine and into morphine, which binds to μ-opioid receptors. Most of the morphine is further converted to morphine-3-glucuronide (M3G; approximately 50%) and morphine-6-glucuronide (M6G; approximately 10%). Recent studies have demonstrated that the brain, pancreas, and myocardium can also produce morphine.
Morphine is metabolized primarily into morphine-3-glucuronide and morphine-6-glucuronide via glucuronidation catalyzed by the liver enzyme UDP glucuronosyl transferase 2B7 (UGT2B7). Morphine is similarly metabolized in brain and kidneys. At least in studies using rodent models, morphine-6-glucuronide is far more potent an analgesic than morphine itself. However, this morphine metabolite crosses the blood-brain barrier poorly in contrast to morphine.
Toxicology
The psychological dependence associated with opioidergic addiction is both protracted and complex. Well beyond the recovery from the physical need for the drug, the opiate addict may obsess about use of the drug and feel an inability to deal with daily activities without use of the drug. These individuals are at high risk for relapse assuming neither the physical environment.
Opiate withdrawal symptoms in opiate users can be seen as early as 2–3 hours after the last dose of drug. Major withdrawal signs peak between 48 to 72 hours after last dose and subside within 9 to 12 days. Initial signs of withdrawal include dilated pupils, profuse sweating, and anxiety. These individuals also experience progressively stronger drug craving, severe abdominal distress, diarrhea, nausea and vomiting, restlessness, muscle and bone pain, insomnia, cold flashes with goose bumps (“cold turkey”) alternating with high temperature spiking (“hot flashes”), and kicking movements (“kicking the habit”). Severe depression is a common manifestation. Opiate withdrawal is rarely fatal, although sudden withdrawal by heavily dependent users in poor health may be fatal. Opiate withdrawal is considered less risky than alcohol, benzodiazepine, or barbiturate withdrawal.
Following intravenous infusion of heroin, the user experiences a surge of euphoria (“rush”) along with dry mouth, a warm flushing of the skin, and a heaviness of the extremities. The user then becomes alternately somnolent and alert (“on the nod”), shifting between wakeful and drowsy states. Mental functioning declines. Tolerance to the effects of the drug develop over time and regular use. In effect, the user must use more heroin to achieve the same intensity of effect. Eventually, drug-induced neural plasticity within reward pathways of the brain results in addiction to the drug.
It is very difficult to establish a lethal dose50 (LD 50 ) for heroin among regular users. Individuals have overdosed on as little as 1 mg/kg of heroin. An LD 50 for nonaddicts has been suggested as 1–5 mg/kg. However, there may be no easily identifiable upper limit to the amount of heroin a heavily addicted individual can take. Research studies conducted in the 1920s in opiate addicts described administering heroin in doses of 1600 to 1800 mg with no obvious adverse side effects. These results are supported by studies in rats showing that 14 days of pretreatment with morphine or heroin reduced mortality associated with subsequent morphine administration. Thus long-term opiate abuse is typically associated with development of highly significant drug tolerance.
Neurological complications associated with heroin use include peripheral neuropathies, nerve pressure palsies, hypoxic encephalopathy, seizures, rhabdomyolysis, and transverse myelopathies. Spongiform leukoencephalopathy is a relatively rare complication of heroin use, with only 70 reported cases from the first reported case in 1984 through 2004. This complication is typically seen among users who inhale fumes generated by heating heroin, a practice called “chasing the dragon.” The typical lesions include abnormal white matter with patchy spongiform change and prominent reactive fibrous gliosis consisting of glial fibrillary acidic protein- (GFAP-)positive fibrous astrocytes. Symptoms vary according the brain regions involved but often include cognitive dysfunction, cerebellar ataxia, dysarthria, and motor restlessness with an estimated mortality rate of approximately 25%.
Heroin-related death has been associated with the phenomenon of place tolerance and overdose as a result of using the drug in an unaccustomed environment. The mechanism for fatal overdosing in this situation was described as an overriding of conditioned or place tolerance. Because heroin use is such a highly ritualized behavior, long-time users exhibit increased tolerance to the drug in locations in which they have repeatedly administered heroin. When they used in a different location, this environment-conditioned tolerance does not occur, which produces enhanced effects of the drug. In response to this decrease in tolerance, the user increases the typical dose of the drug. If extreme in self-dosing, the result can be a fatal overdose.
Morphine also affects immune system function via interactions with dendritic cells. Dendritic cells, a type of antigen-presenting cell, express opiate receptors. Dendritic cells exposed to morphine during their maturation produce increased levels of interleukin-12, a cytokine responsible for promoting the proliferation, growth, and differentiation of T lymphocytes, which are active in adaptive immune responses and less so, interleukin-10, a cytokine responsible for promoting B-lymphocyte responsiveness.
Hallucinogens
History
Hallucinogens comprise a class of drugs defined by their ability to induce changes in the user’s perception of reality. Users describe seemingly real images, sounds, and sensations that do not in fact exist. Traditionally, these drugs were derived from plant sources but are now synthetic with resultant greater purity of product (Drug Enforcement Administration, Drug Descriptions: Hallucinogens). Commonly used hallucinogens include lysergic acid diethylamide (LSD) and psilocybin ( Fig. 17.7 ).

Lysergic acid is a component of ergot alkaloids found in the ergot fungus ( Claviceps purpurea ), which infects cereal grains including rye. Ergot alkaloids are very potent compounds responsible for ergotism (also known as ergotoxicosis, ergot poisoning, and St. Anthony’s Fire) characterized by convulsive and vasoconstrictive symptoms including gangrene and hallucinations, mania and psychoses.
Lysergic acid diethylamide (or LSD) was first synthesized in 1938 by Swiss chemist Albert Hofmann (1905–2008). The hallucinogenic effects of LSD were not immediately recognized and the drug was ignored over the next several years. But in 1943, Hofmann accidentally absorbed a very small amount of LSD and experienced first-hand the psychogenic effects of the drug. He then followed up with an additional self-administration of LSD, later reporting the intensely psychedelic effects associated with the drug. The abbreviation LSD is derived from its early codename LSD-25 (German “lysergsäure-diethylamid” followed by a sequential development number). LSD was initially marketed by Sandoz Laboratories as a therapeutic drug with numerous potentially useful psychiatric applications. However, the drug’s entry into the illicit, recreational environment with ensuing political repercussions eventually precluded further interest in the drug among pharmaceutical companies and physicians. The substance was eventually banned for any use other than research as a DEA Schedule I substance.
For a time during the 1950s and early 1960s, intelligence agencies in the United States and other countries had an interest in use of LSD to facilitate interrogations and mind control. The Central Intelligence Agency (CIA) conducted such studies through the Office of Scientific Intelligence largely in response to similar studies employed by the Soviet Union and China in the early 1950s. One such study involved the use of US soldiers dosed with LSD to study the effects of panic. By the late 1960s the CIA had ended these LSD studies, the results of which were considered too unpredictable.
The principal psychoactive component of so-called magic mushrooms is an indole related to tryptamine, psilocybin. Use of hallucinogenic mushrooms (Psilocybe cubensis and P. semilanceata ) may go back as far as prehistoric humans, although conclusive evidence for this is lacking. Their early use was probably associated with religious communing, divination, and healing just as they are among some present-day Native Americans. Bernardino de Sahagún (1499–1590), a Franciscan missionary, described the ritualistic use of teonanácatl or “flesh of the gods” among the Central American Aztecs. In the 1960s, recreational use of hallucinogenic mushrooms was promoted by R. Gordon and Valentina Wasson, Timothy Leary, and others, which led to the popularization of a number of psychoactive Psilocybe species found in North America, Europe, and Asia.
In 1958, Hofmann had identified psilocin and psilocybin as the active compounds in psychoactive mushrooms. By the early 1970s, a number of psychoactive Psilocybe species were described, these variants being found in North America, Europe, and Asia. These mushrooms may also contain small amounts of other psychoactive tryptamines. Psilocybin and psilocin are listed as Schedule I drugs in the United States and many other countries ( Fig. 17.8 ).

Mushroom concentrations of psilocybin and psilocin vary significantly among varieties of psychoactive mushrooms but averages 0.5%–2.0% of the dry weight of the average mushroom. The more common species P. cubensis contains approximately 10–25 mg psilocybin and psilocin. When psilocybin is ingested, it is broken down to produce psilocin, which is responsible for the hallucinogenic effects. Twenty-five milligrams to 50 mg of psilocybin and/or psilocin is generally thought to be a heavy psychoactive dose of these drugs.
Pharmacodynamics
Mechanisms of Action
The hallucinogenic effects of LSD and phenethylamine hallucinogens are thought to be mediated by the binding of these drugs to the 5HT 2A receptor. LSD administered in so-called recreational doses has been shown to interact with 5-HT 1A , 5-HT 2A , 5-HT 2C , 5-HT 5A , 5-HT 5B , and 5-HT 6 receptors. More specifically, the hallucinogenic effects of LSD have been attributed to the drug’s strong partial agonist effects at 5-HT 2A receptors ; selective 5-HT 2A specific antagonists block the psychotropic activity of LSD. With the exception of the ligand-gated ion channel 5-HT 3 receptor, all other 5-HT receptors are G-protein-coupled seven transmembrane receptors that activate intracellular second messenger pathways. The exact sites and mechanisms of action and are not yet known. In addition to the drug’s effects on 5HT 2A receptors, LSD has been shown to affect glutaminergic systems within the CNS. Systemic LSD (0.1 mg/kg, i.p.) or direct LSD infusion (10 μM) into prefrontal cortex has been associated with elevated levels of glutamate release in this brain region, an effect blocked by administration of the 5HT 2A antagonist M100907 (0.05 mg/kg, i.p.). Chronically, LSD may activate dopamine- and cyclic AMP-regulated phosphoprotein with molecular weight 32 kDa (DARPP-32)–related pathways, a mechanism of action shared by numerous other psychoactive drugs including cocaine, amphetamine, methamphetamine, nicotine, caffeine, LSD, PCP, ethanol, and morphine.
Typical doses of LSD are measured in microgram amounts rather than the more typical milligram amounts associated with other drugs of abuse. Hofmann determined that an active dose of mescaline, roughly 0.2 to 0.5 g, has effects comparable to 100 μg or less of LSD. A single dose of LSD is typically 100 to 500 μg; threshold psychotropic effects of the drug experienced with as little as 25 μg. . The LD 50 for LSD has been estimated to range from 200 μg/kg to more than 1 mg/kg, although there are no known deaths attributed directly to the use of LSD. LSD users do not exhibit the typical features of drug addiction and dependence; however, tolerance to the drug can develop rapidly. Users demonstrate cross-tolerance between LSD and psilocybin. Attenuation of tolerance to LSD is thought to be related to drug-induced downregulation of 5HT 2A receptors in as yet undefined CNS areas.
Adverse reactions to LSD have been treated using fast-acting benzodiazepines such as diazepam or triazolam. These serve as anxiolytics, calming the patient but without directly blocking LSD binding at 5HT 2A sites. Theoretically, specific 5-HT 2A receptor antagonists, for example, the atypical antipsychotic quetiapine fumarate, would act to block LSD binding at these receptors, thus attenuating the psychoactive effects of LSD.
Mescaline (3,4,5-trimethoxyphenethylamine) is a naturally occurring hallucinogenic phenethylamine. Mescaline is one of several psychoactive alkaloids produced by several species of cactus including the peyote cactus ( Lophophora williamsii ), the San Pedro cactus ( Echinopsis pachanoi ), and the Peruvian Torch cactus ( Echinopsis peruviana ). The peyote cacti are primarily subterranean, with underground roots and a relatively small above-ground crown consisting of several disk-shaped “buttons.” These buttons are cut from the cactus and dried. Peyote includes a number of alkaloids including mescaline. Peyote has been used as a part of religious rites by Native American Indians of the arid northern Mexico and southwest United States for thousands of years. Peyote buttons with measurable levels of mescaline were found within prehistoric native Indian ruins and traced back to 3780–3660 BCE by radiocarbon dating. Mescaline was first isolated and identified in 1897 by German chemist Arthur Heffter and first synthesized in 1919 by Ernst Späth ( Fig. 17.9 ).

Mescaline is rapidly absorbed after oral ingestion by rats. In human, the effective dose range is 300–500 mg of pure mescaline. The hallucinogenic effects of associated with ingestion of mescaline are seen in doses of 300–600 mg, the equivalent of 9–20 small peyote cactus tops. Mescaline is 1000–3000 times less potent than LSD, and 30 times less potent than psilocybin. The LD 50 has been estimated as 212 mg/kg, i.p., for mice; 132 mg/kg, i.p., for rats; and 328 mg/kg, i.p., for guinea pigs. About half the initial dosage is excreted after 6 hours, but some studies suggest that it is not metabolized at all before excretion. The effects of mescaline can last up to 12 hours. Tolerance to mescaline increases with repeated administration. Mescaline may exhibit cross-tolerance with either LSD or psilocin. Equipotent doses of mescaline and LSD have been described as all but indistinguishable in psychoactivity. A significant amount (20%–50%) of an ingested dose of mescaline is excreted in the urine unchanged in canine experimental models. Lesser amounts (7%) are excreted in urine by humans.
In contrast to LSD-induced hallucinations, those associated with mescaline use are described as being consistent with actual experiences but are typically intensified through visual and auditory inputs. Mescaline elicits a pattern of sympathetic arousal, with the peripheral nervous system being a major target for this drug. Similar to LSD, mescaline binds to and activates brain serotonin 5-HT 2A receptors with a high nanomolar affinity.
Pharmacokinetics
Routes of Administration
LSD is typically administered orally. Often absorbent paper, sugar cubes, or gelatin cubes are used as vehicles to deliver very small amounts of the drug. Unlike most other medicinal or illicit drugs dosed in milligram concentrations, psychoactive doses are measured in microgram concentrations. Liquid forms of the drug can be administered either intramuscularly or intravenously. Twenty micrograms to 30 μg is thought to be a threshold dose to experience psychoactive effects. The psychoactive effects of a threshold dose (20–30 μg) of LSD typically last from 6–12 hours depending on tolerance, body weight, and age. These effects do not last longer than measurable blood levels of LSD as was once thought. Aghajanian and Bing reported that LSD had an elimination half-life of 175 minutes. In a case study involving a single adult male, a 1 μg/kg dose of LSD orally had a plasma half-life of 5.1 hours, with a peak plasma concentration of 5 ng/mL 3 hours after drug administration. These investigators also reported a close correlation between measurable blood concentrations of LSD and the time course of the subject’s difficulties with simple arithmetic problems. Recent research suggests that LSD binds to the 5-HT 2A receptor in such a way that locks it into the receptor binding site, preventing rapid dissociation of the molecule and potentially leading to prolonged action. Following ingestion, psilocybin is rapidly absorbed and dephosphorylated to psilocin. Similar to LSD, psilocin is a highly potent 5HT 1A , 5-HT 2A , and 5-HT 2C receptor agonist. The receptor binding potency of psilocin correlates strongly with its potency as a hallucinogen. The psychoactive effects of psilocin can be highly variable among individuals. Effects reported by many individuals include strong visual and auditory components. Ingestion of psilocybin and/or psilocin is associated with an increase in the ability to concentrate on memories, feelings of time expansion, abstract and distractive thought patterns, as well as indecisiveness, phonetic experimentation (glossolalia), and epiphanies about life.
Psilocybin has a reported onset of action of 15–30 minutes following ingestion, with psychoactive effects lasting 5–8 hours. The duration of psychoactive effects correlates with dosage, itself a function of mushroom preparation and storage, and with variations in metabolism among users.
Toxicology
LSD has been shown to bind to and induce conformational changes in the structure of the DNA helix. And although LSD has been reported to be mutagenic at higher doses in animal models, no detectable DNA damage or increased incidence of cancers has been seen with LSD use in humans.
In fact, most hallucinogens are not known to have long-term toxicities. However, an important caveat is the potential for MDMA to produce free radicals as a side reaction to the effects of this drug on biogenic amine systems in the CNS. These free radicals may induce neurodegeneration within various brain areas with resultant disease states. Hallucinogen persisting perception disorder (HPPD; Fourth Edition of the Diagnostic and Statistical Manual of Mental Disorders [DSM-IV] diagnosis: diagnostic code 292.89) represents a condition in which the vision system-related effects of drug persist over a long period of time. HPPD is distinctly different from so-called “flashbacks” in being persistent. The mechanism for this disorder has not been defined.
To date no significant toxicities have been associated with ingestion of psilocybin mushrooms. However, a lethal dose in humans has not been established. The oral LD 50 in rats is 280 mg/kg. Psilocybin represents approximately 1% of the dried weight of the P. cubensis mushroom. An adult weighing 60 kg would have to ingest 1.7 kg of dried mushrooms to reach a dosage equivalent to the oral LD 50 in rats. Psilocybin and psilocin are not considered addictive, although both can induce short-term increases in tolerance of users.
Therapeutic Use
Recent research suggests that hallucinogens might be used therapeutically in some situations. Results of clinical trials have shown positive effects of MDMA in depression and posttraumatic stress disorder, and as an anxiolytic for terminally ill patients. Recent pilot studies have even examined the potential of psilocybin to treat addiction to tobacco and alcohol.
Cannabis
History
In 1378, the Emir of the Joneima in Arabia, Soudoun Sheikouni issued the first recorded edict prohibiting cannabis use. He ordered all cannabis plants in the region destroyed and that those convicted of ingesting the plant have all of their teeth removed. Fewer than 20 years later, in 1393, use of cannabis in Arabia had increased. And so it goes even today with the allure of this unique plant. Despite centuries of government edicts from all corners of the globe, cannabis remains the most popular psychoactive substance on the planet with the exception of caffeine, tobacco, and ethanol.
Cannabis has been used in China for over 5000 years. Its use in the Middle East is probably similarly ancient. The translation of the ingredients of the holy oil used by Aaron and his sons to anoint the tabernacle of Moses consisted of myrrh, cinnamon, cassia (commonly used as cinnamon in North America), and calamus extracted into olive oil. However, in the original Hebrew text, the last ingredient is “kanah bosm,” which some contend is actually the Sycthian etymological root of cannabis. Indeed, the Greek historian Herodatus describes recreational use of cannabis among the Sycthians 2500–3000 years ago.
Cannabis is the genus name given to several strains of the plant commonly called hemp. As early as 1855, it was recognized that hemp carefully cultivated in the gardens of the Near and Far East had vastly different properties when consumed than the hemp grown as a large scale crop in Europe, which was used in the production of fibers for rope, paper, and fabric. For thousands of years in the Near and Far East, preparations of cannabis were smoked, eaten, or prepared in beverages. Thus although improvements in refining and distilling capabilities over the past 200 years have led to drastic increases in the potency and portability of drugs such as cocaine, morphine, and even ethanol, cannabis users continue to employ the same methods practiced by prehistoric peoples. However, modern methods of drug preparation, including extraction with carbon dioxide or solvents can produce a more concentrated product. 325 More recently, synthetic chemicals that mimic the effects of cannabis (synthetic cannabinoids) have gained popularity as recreational drugs. The first synthetic cannabinoids identified were developed by Roger Adams in the mid-20th century. Over the next several decades, additional synthetic cannabinoids were developed; however, these molecules remained confined largely to research laboratories. In the mid-2000s, a team of chemists led by John Huffman synthesized a series of synthetic cannabinoids, with the goal of producing peripherally restricted cannabinoids with little or no psychoactive activity. However, several of the molecules they synthesized had substantial psychoactive activity. The relatively simple synthesis and availability of precursors, combined with their legal status, resulted in large-scale production by international chemical laboratories. These chemicals continue to be incorporated into recreational drug products and sold worldwide as recreational drug products.
These chemicals are derived from several different chemical classes, and are broadly described as synthetic cannabinoids. Because manufacturers are continuously altering the chemical structure, these drugs are often not detected using common drug test methods. Emerging evidence indicates that these chemicals are more dangerous than phytocannabinoids produced by the cannabis plant, perhaps due to their greater pharmacological potency and efficacy.
Chemical Properties
Raw cannabis contains 483 distinct chemical constituents, most of which are common to other plants. However, the genus Cannabis alone produces the 66 known chemicals that constitute the cannabinoids. Cannabinoids are terpenes joined to an alkyl-substituted resorcinol. Several of the cannabinoids are psychoactive, most notably Δ -tetrahydrocannabinol (THC; see Fig. 17.1 ). THC is regarded as the principal psychoactive constituent of cannabis, and can produce discriminative stimulus effects in experienced cannabis users. THC has a molecular weight of 314. It is insoluble in water, and experimental preparations commonly employ the use of an emulsifier such as vegetable oil to allow an injectable solution. The concentration of THC in cannabis depends upon the source, with levels ranging from 0.007% to almost 4.0%. Although official reports released by the US Department of Justice assert that the concentration of THC in cannabis is increasing both in the United States and abroad, others, including the director of the Univ of Mississippi Marijuana Potency Monitoring Project Mohammed ElSohly, dispute this claim ( http://www.slate.com/?id=2074151 ). Selective breeding techniques have undoubtedly resulted in enriched THC-containing strains, notably in Canada and The Netherlands; however, after several generations in the United States, the THC content of these strains recedes to levels common to American plants. Climate, light, soil, humidity, and stress during the growing season all affect THC content.
Synthetic cannabinoids have been derived from at least five different chemical classes. These include classical cannabinoids, which share structural similarities with phytocannabinoids; nonclassical cannabinoids, which are similar to classical cannabinoids, but lack a third fused ring; aminoalkylindoles, which are structurally diverse and represent the most common synthetic cannabinoids used recreationally; eicosanoids, which are fatty acids that serve as endogenous cannabinoids in mammals; and others that are often chimeras from the other four classes. Generally these compounds share the lipophilicity of phytocannabinoids. Thus preparation of these chemicals typically involves dissolving them in a solvent, and then applying the solution to nonpsychoactive plant material such as potpourri. Once the solvent evaporates, the synthetic cannabinoid-impregnated product is packaged and sold, to be smoked similar to cannabis by the consumer. Although the raw material obtained from chemical suppliers is relatively pure, this production results in widely variable concentrations, even within the same package. Furthermore, synthetic marijuana products often contain several different synthetic cannabinoid molecules. These factors make predicting the quality and intensity of the effect of these drugs highly unpredictable.
Pharmacokinetics
Routes of Administration
Marijuana is most commonly smoked. The plant material is macerated and rolled into cigarettes or loaded into a pipe. Some people utilize a water pipe in which the smoke is drawn through water, with the intent of removing toxic compounds resulting from pyrolysis. This method does appear to effectively reduce the ingestion of pyrolytic toxins. However, a study funded by the Multidisciplinary Association for Psychedelic Studies (MAPS) and the California chapter of the National Organization to Reform Marijuana Laws (CaNORML) showed that while water pipes do filter out tar, the water also traps substantial amounts of THC, which leads the user to ingest more smoke, offsetting the benefits of water filtration. An alternative to smoking that is growing in popularity is vaporization. This technique requires specialized equipment that heats the plant material up to 200°C, the vaporization temperature of THC and related compounds, but not hot enough to result in combustion. This method has been shown to result in similar subjective effects (almost 90% of the vaporized substance is THC), yet almost completely eliminate combustion byproducts in the inhaled product.
THC can also be eaten. Typically, fat-soluble cannabinoids are extracted into butter or some other oil, which is filtered and used to make foods. Although this method eliminates any byproducts of combustion, the onset of psychoactive effects is slower and more difficult for the user to titrate. Alternatively, cannabinoids can be extracted from the plant material with ethanol. The ethanol can then be consumed or used as a tincture. Again, this method eliminates harmful byproducts resulting from combustion, but makes dose titration more difficult. Furthermore, impairment due to cannabis is enhanced by ethanol, possibly due to pharmacokinetic or pharmacodynamic interactions. Oral and topical preparations were the most commonly used medicinal applications in the late 19th and early 20th centuries.
Another preparation, hashish, has been used for centuries in some regions. Hashish describes a preparation of the resin (which contains a high concentration of the psychoactive constituents in cannabis. More recently, THC extract in a liquid or wax-based form has gained popularity. This can be accomplished by soaking raw cannabis in solvent (often butane) or exposing it to pressurized CO 2 . The resulting product is a waxy substance with extremely high THC content. 325
Synthetic cannabinoids can also be prepared in a liquid vehicle, often a propylene glycol/vegetable oil mixture, similar to the vehicle used in electronic cigarettes to deliver nicotine. This preparation can be used in the same types of vaporizer pens used to consume liquid nicotine preparations ( Fig. 17.10 ).
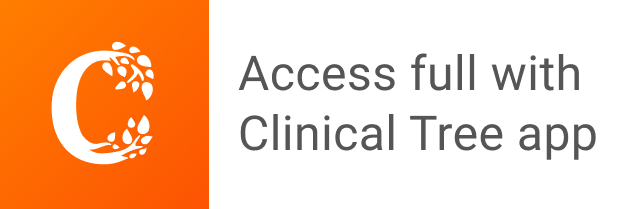