7 Lawrence H. Lash This chapter discusses various aspects of renal toxicology. To put the subject into its proper context, it is critical to understand the morphological, functional, and biochemical features of the kidneys that influence or largely determine the susceptibility of this organ to chemically induced toxicity. Thus, Section 7.1 summarizes some key aspects of renal structure and function and describes how these properties impact or determine the toxic responses of the kidneys to chemical or drug exposures. Section 7.2 describes the major classifications of both acute and chronic renal injury and summarizes some key aspects of how renal cells respond to these forms of injury at the cellular and molecular levels. While this chapter will by no means be a treatise on the cellular and molecular mechanisms by which nephrotoxic chemicals act, it will highlight some of the major mechanisms by which chemicals act and give an indication of which ones have been modulated to develop novel therapeutic approaches to treat nephrotoxicity. Section 7.3 summarizes common methods that are used to assess renal function and toxicity in clinical settings and in the whole animal. Section 7.4 discusses some of the most commonly used in vitro models from both laboratory animals and human kidney tissue that are used to screen chemicals for nephrotoxicity or investigate their mechanism of action. Finally, Section 7.5 provides some examples of nephrotoxic chemicals, and includes both environmental contaminants and therapeutically used drugs. Mammals possess two kidneys located near the lower ribs. Each kidney receives its blood supply from a single renal artery that originates in the aorta. Renal blood drains into a single renal vein that connects with the inferior vena cava. Urine normally empties from a single ureter and then into the bladder. On a gross, whole-organ level, the kidneys are subdivided into three regions: cortex, outer medulla, and inner medulla. The outer medulla is further divided into the outer stripe and inner stripe. The inner medulla is further divided into the base and tip; the latter is also called the papilla. Whereas small mammals, such as the rat, mouse, and rabbit, have a single papilla in each kidney, larger mammals, including humans, have multiple papilla per kidney. In either unipapillate or multipapillate mammals, urine exits from the tip of the papilla into the renal pelvis. The functional unit of the kidneys is the nephron (Figure 7.1), which is a series of epithelial cells of varying types (see later) that form a tube of one-layer-thick cells enclosing a luminal compartment. Mammalian kidneys contain millions of nephrons, each of which leads into a common collecting duct. Nephrons are categorized as either superficial, midcortical, or juxtamedullary, depending on the location of their glomeruli. The renal cortex contains glomeruli, proximal convoluted and some proximal straight tubules, macula densa, cortical thick ascending limbs, distal convoluted tubules, connecting tubules, initial collecting ducts, interlobular arteries, and afferent and efferent capillary networks. The outer stripe of the outer medulla is located just below or inside the cortex and contains proximal straight tubules, medullary thick ascending limbs (mTAL), and outer medullary collecting ducts. The inner stripe of the outer medulla is located below or inside the outer stripe and contains thin descending limbs, thick ascending limbs, and outer medullary collecting ducts. The inner medulla contains thin descending limbs, thin ascending limbs, and inner medullary collecting ducts. The renal vasculature surrounds the tubule and parallels the nephron. Figure 7.1 Schematic of the basic functional unit of the kidneys, the nephron. The scheme illustrates the basic functional unit of the kidneys, the nephron, and shows the parallel renal vasculature, the major nephron segments, and the common collecting duct into which each nephron empties. The primary function of the kidneys is to maintain the internal environment of the body. They do this by regulating total body salt (e.g., sodium, potassium, and chloride ions), water, and acid–base balance (e.g., proton, bicarbonate, and ammonia transport and metabolism), reabsorbing nutrients (e.g., glucose, amino acids), and excreting waste products (e.g., urea, creatinine, uric acid, phosphates, sulfates). As shown in Table 7.1, the kidneys are extremely efficient at reabsorbing key electrolytes and water. The critical function of the kidneys in water conservation is exemplified by a consideration of how fluid input and output are balanced. Water input is determined largely (~88%) by ingestion via the gastrointestinal tract, with the remainder (~12%) coming from metabolism. In contrast, water output is determined primarily by the kidneys (~72%), with the remainder coming from the skin (~12%), lungs (~12%), and gastrointestinal tract (~4%). Table 7.1 Filtration and Reabsorption of Water and Electrolytes by the Human Kidney Three general mechanisms are involved in accomplishing these key functions: glomerular filtration, tubular reabsorption, and tubular secretion. Glomerular filtration is defined as the movement of water and solutes across the glomerular capillary wall to form an ultrafiltrate of plasma. The rate at which this process occurs is called the glomerular filtration rate (GFR). A typical GFR in a normal, young, healthy adult human is 125 ml/min or 180 l/24 h. As discussed in Section 7.3, this GFR value represents a high degree of reserve function such that individuals are not considered to have compromised renal function until GFR declines to below 60 ml/min. The movement of electrolytes or metabolites from the tubular fluid back into the plasma is called tubular reabsorption, resulting in retention in the body, whereas that from plasma into the tubular fluid is called tubular secretion, ultimately resulting in excretion into the urine. The kidneys also possess specialized endocrine functions, such as synthesis of 1,25-dihydroxy vitamin D3 and the protein erythropoietin. The latter is critical for synthesis of red blood cells. In fact, deficiency in the renal synthesis of erythropoietin is invariably associated with anemia. Additionally, although the liver is considered the primary organ of drug metabolism, the kidneys possess significant drug metabolism capabilities. As described in Section 7.5, some classes of chemicals are specifically bioactivated by renal enzymes to reactive metabolites that lead to their nephrotoxicity. The mammalian kidney is a complex organ whose basic structural unit, the nephron, is composed of many cell populations, each exhibiting diverse morphological, biochemical, and functional properties (Table 7.2). Differences exist among cell types in morphology, membrane structure, energetics, and the composition of transporters and drug metabolism enzymes. As a consequence of this functional heterogeneity, exposure of the kidneys, either in vivo or in vitro, to various nephrotoxicants or to pathological conditions such as ischemia or hypoxia, produces distinct patterns of cellular injury. Thus, certain cell populations are either specific targets of or are particularly susceptible to one form of injury or another. In some cases, the specificity is due to the selective presence in a given cell population of a membrane transport system or a bioactivation or detoxication enzyme. In other cases, however, susceptibility is explained by the basic biochemical function of the cell. Table 7.2 Differential Morphology, Biochemistry, and Physiology of Selected Nephron Segments BB, brush-border; BL, basolateral; CYP, cytochrome P450; DCT, distal convoluted tubule; FA, fatty acid; FMO, flavin-containing monooxygenase; GSH, glutathione; mTAL, medullary thick ascending limb; OA–, organic anion; OC+, organic cation; OM, outer medulla; OSOM, outer stripe of outer medulla; OXPHOS, oxidative phosphorylation; PGS, prostaglandin synthase. For example, mTAL cells are particularly susceptible to injury from hypoxia or ischemia–reperfusion because of their relatively poor oxygenation and primary reliance on glycolysis for generation of ATP. The proximal tubules are the most common targets for chemically induced injury because they are the first nephron cell type with which filtered chemicals come in contact, they possess a large array of membrane transporters that mediate uptake and intracellular accumulation of chemicals (see later), and they contain relatively high activities of drug metabolism enzymes that can metabolize chemicals to reactive and toxic species. The ability to generate the high osmolarity of the medullary interstitium is dependent on two principal factors: (1) active chloride or salt reabsorption in the ascending limb, and (2) the anatomical relationship between the descending and ascending limbs of the loop of Henle; the fact that both limbs are close together and that flow in each limb is in the opposite direction is a critical feature. This results in what is called countercurrent flow. Additionally, as noted earlier, there is a parallel vascular structure to the nephron structure that forms a countercurrent exchange of blood. This arrangement prevents solutes from coming out in the descending limb and being washed away but also contributes to the ability of the renal epithelium to concentrate certain chemicals to levels several-fold higher than those in either the plasma or glomerular filtrate. A critical feature of all transporting epithelia is the presence of an array of carrier proteins selectively localized on either the brush-border plasma membrane (BBM) or basolateral plasma membrane (BLM) that mediates the uptake and/or efflux of ions and metabolites. Because of nephron heterogeneity, most of the transport of drugs and environmental chemicals occurs in the proximal tubules as these cells contain the highest activities of carrier proteins for organic anions (OA–) and cations and intermediary metabolites. Three modes of transport are relevant to a consideration of the role of carrier-mediated transport in chemically induced nephrotoxicity (Figure 7.2). In the pathway for chemical A, the chemical is taken up into the cell from the lumen by transport across the BBM and is then reabsorbed into plasma by efflux across the BLM. In contrast, in the pathway for chemical B, the chemical is taken up into the cell from the plasma by transport across the BLM and is then excreted into the lumen and ultimately the urine by efflux across the BBM. For the pathway for chemical C, however, high-affinity uptake from plasma occurs across the BLM. Poor transport across the BBM, however, leads to intracellular accumulation of chemical C, thereby promoting toxicity. Figure 7.2 Modes by which plasma membrane transport determines drug disposition and toxicity in the renal proximal tubule (PT). Pathway A illustrates reabsorption of filtered chemicals by uptake into the PT cell across the brush-border plasma membrane (BBM) and then efflux from the cell into the renal plasma and interstitial space by transport across the basolateral plasma membrane (BLM). Pathway B illustrates secretion of chemicals from the renal plasma and interstitial space by successive transport across the BLM and then the BBM; the effluxed chemical is then secreted into the urine. Pathway C illustrates how chemicals in the renal plasma and interstitial space are transported into the cell across the BLM and, due to relatively poor transport out of the cell into the tubular lumen, how the chemical can accumulate in renal cells thereby enhancing toxicity. The high efficiency by which renal cells, particularly those of the proximal tubule, take up chemicals from the plasma is associated with the presence of coupled, active transport systems in the plasma membranes (Figure 7.3). This coupling is achieved on the BLM by the action of the primary active transporter the (Na+ + K+)-stimulated ATPase, which catalyzes efflux of two Na+ ions in exchange for uptake of three K+ ions and is energized by the hydrolysis of ATP. This primary active transporter, so called because it uses high-energy ATP, generates a large, inwardly directed gradient of Na+ ions across the BLM that can be used to facilitate uptake of anions. Such a facilitated, Na+-coupled uptake occurs for 2-oxoglutarate (2-OG2–), which is catalyzed by the sodium dicarboxylate 3 carrier (NaC3; SLC13A3). This is considered a secondary active transporter as it uses the Na+ gradient generated by a primary active transporter to facilitate uptake of other substrates. The NaC3 carrier, in turn, generates an inwardly directed gradient of 2-OG2–, which is used in a tertiary active transport process to catalyze uptake of OA–, which can be mediated by, among others, the OA– transporters 1 and 3 (OAT1/3; SLC22A6/8). Figure 7.3 Coupling of primary, secondary, and tertiary active transport systems on the basolateral plasma membrane (BLM) of the renal proximal tubule. Metabolite (M(–)) transport across the BLM is ultimately energized by direct or indirect coupling to ATP hydrolysis or coupling to ion or metabolite gradients generated by ATP hydrolysis. The (Na+ + K+)-stimulated ATPase is considered a primary active transporter as it generates Na+ and K+ electrochemical gradients by using the energy from ATP hydrolysis. Na+ ions are then coupled to uptake of metabolites or 2-oxoglutarate (2-OG2–), the latter being mediated by the sodium dicarboxylate carrier 3 (NaC3; Slc13a3). The NaC3 generates an electrochemical gradient of 2-OG2–, which is then coupled to the uptake of organic anions (OA–), primarily by the OA– transporter 1 and 3 (Oat1/3; Slc22a6/8). Inasmuch as Oat1 and Oat3 are energized by the 2-OG2– gradient, which is in turn generated by the NaC3, they are termed tertiary active transporters. The importance of these coupled processes is highlighted by the realization that a substantial number of drugs and nephrotoxicants are OA– and are thus transported across the BLM by OAT1 and/or OAT3. These carriers, particularly OAT1, are inhibited by the classic OA– transport inhibitor probenecid. For many nephrotoxicants that are OA–, their toxicity can be modulated by probenecid, demonstrating the critical role of the BLM transport step. Coadministration of multiple drugs that are all transported by these anion carriers can lead to drug–drug interactions that limit the therapeutic efficacy of these drugs and may lead to nephrotoxicity as a side effect of exposure. The foregoing discussion highlights the various aspects of renal physiology that contribute to susceptibility of the kidneys as a target organ for many toxic chemicals. These factors are summarized in Table 7.3. As described earlier, the physiological functions of the kidneys can enhance nephrotoxicity when the chemical being handled is a nephrotoxicant. Additionally, membrane transporters and drug metabolism enzymes that are similar to those found in the liver, which is the organ usually considered the major site of drug metabolism, also exist in the kidneys, particularly in the proximal tubules. Table 7.3 Factors Influencing Susceptibility of Kidneys to Chemically Induced Nephrotoxicity In considering the manner by which chemicals can produce kidney injury, critical aspects include the dose and exposure time. This is a general point that applies not only to exposure to nephrotoxicants, but to exposure to any toxicant and any target organ. The importance of this is that exposures to relatively high doses of chemicals as a single dose or multiple doses over short periods of time (acute exposures) and those to relatively low doses of chemicals over longer periods of time (chronic exposures) result in fundamentally different organ responses. There are four basic mechanisms by which chemicals can produce acute kidney injury: Besides chemically induced carcinogenesis, chronic exposures to nephrotoxic chemicals produce renal injury primarily by two mechanisms, chronic tubulointerstitial fibrosis and papillary necrosis. Analgesics and other NSAIDs are linked to these mechanisms. The former, like acute tubulointerstitial fibrosis, involves immunological and inflammatory effects. The latter mechanism, papillary necrosis, occurs because the patterns of renal blood flow over time lead to accumulation of drug in the renal medulla. Exposure to a nephrotoxicant can result in a broad range of responses (Figure 7.4). The precise response will vary due to four general factors: (1) the nature of the chemical, (2) exposure dose, (3) exposure duration or pattern, and (4) other factors that may alter susceptibility to renal injury. Figure 7.4 Generalized scheme of responses of renal cells to nephrotoxicant exposures. Upon exposure to potentially nephrotoxic chemicals or drugs, renal cells elicit a range of responses, largely dependent on the dose and duration of the exposure. Exposure to high concentrations of nephrotoxicant typically leads to cytotoxicity, which can result in acute tubular necrosis or apoptosis, although the renal proximal tubular cell can also repair sublethal injury. Exposure to relatively low doses of nephrotoxicant can produce more subtle effects, such as changes in gene expression or signal transduction pathways. This may ultimately lead to tumorigenesis or repair. The repair process typically occurs in three phases: proliferation, reepithelialization, and differentiation. It would seem intuitive that the chemical properties of the nephrotoxicant would play a major role in determining what type of response occurs at the cellular level. Indeed, some chemicals, because of their physicochemical properties (e.g., charge, lipid solubility) or their bioactivation (metabolism) to a specific reactive species, interact with very specific molecular components of the renal cell, thus producing very specific effects. Some of the examples of nephrotoxicants that will be discussed in Section 7.5 will highlight these targeted mechanisms of action. A basic tenet of all Pharmacology and Toxicology is, to quote Paracelsus, that “the dose makes the poison.” Thus, whereas acute cytotoxicity tends to be a response to toxic insult from high-dose exposures, altered cellular regulation more typically occurs at lower doses of toxicant exposures (cf. Figure 7.4). It is also well known that many nephrotoxic chemicals can produce both acute tubular necrosis and apoptosis, with the former occurring at relatively high doses and the latter occurring at relatively low doses. Similar to dose, duration or pattern of exposure are also central components of pharmaco- and toxicokinetics. Chemical exposures can be either acute (continuous and short-term over a period of <2 h or a single exposure), subchronic (continuous or repeated exposures over a period of <3 months), or chronic (continuous or repeated exposures over a period of >3 months). Acute exposures are typically at relatively high doses (e.g., overdose toxicity) whereas chronic exposures are typically at relatively low doses (e.g., exposures to carcinogens that are environmental contaminants). Many other factors may contribute to eliciting different responses to nephrotoxicants in humans. Some of these include prior or simultaneous exposure to other drugs or toxic chemicals, the existence of a disease or pathological state, or genetic polymorphisms in drug metabolism transporters or enzymes. In each case, the susceptibility of the individual is altered, thereby changing the toxic response from what might have been expected in the typical individual. In the first case, for example, prior or simultaneous exposure to other drugs or toxic chemicals may alter the response to the chemical in question because of drug–drug interactions based on such processes as competition for transport, enzyme induction or inhibition, or potentiation of toxic effect. In the second case, the exacerbation of nephrotoxicity by several diseases or pathological states has been well documented. Examples include liver disease, diabetes, and cardiovascular disease. In the third case, genetic polymorphisms may alter the ability of kidney cells to bioactivate or detoxify nephrotoxicants or to absorb or secrete them. Genetic polymorphisms, which are inherited mutations that occur in a population with a frequency of at least 1–2%, result in either altered activity of proteins or even the absence of a protein. Examples of polymorphisms that may be relevant to the ability of the kidneys, particularly the proximal tubular cells, to handle nephrotoxicants include those in drug metabolism enzymes such as cytochrome P450s (CYP) or glutathione S-transferases or in plasma membrane transporters such as the OA– transporter 1 and 3 (OAT1/3; SLC22A6/8) or the various forms of the multidrug resistance proteins (MRPs). In considering the cellular mechanisms by which kidney cells, in particular proximal tubular cells, respond to exposures to nephrotoxicants, it is important to understand that the renal epithelium has a significant capacity to undergo proliferation and recovery (cf. Figure 7.4). When renal epithelial cells undergo repair and proliferation, the newly generated cells are dedifferentiated, meaning that they do not express most of the proteins whose function are characteristic of mature kidney cells. There are two subsequent phases of recovery: Phase I is re-epithelialization, in which gaps in the epithelial monolayer that were caused by cell death are filled in by the new dedifferentiated cells; this is followed by differentiation of these newly generated cells. As noted in Figure 7.4, the altered cellular regulation that arises from the toxic insult may, for some chemicals, cause transformation of the renal epithelial cells to produce tumorigenesis. Additionally, it has been suggested that chronic, repeated exposures to some nephrotoxicants cause repeated cycles of cytotoxicity or altered cellular regulation, followed by repair and proliferation. This in turn leads to tumorigenesis through a so-called epigenetic mechanism. Examination of Figure 7.4 raises the question of how nephrotoxic chemicals produce their toxic insult. There are several possible mechanisms, which are illustrated in Figure 7.5. In general, most nephrotoxic (or just toxic for that matter) chemicals must undergo some form of metabolism or biotransformation to produce metabolites, some of which are called “reactive intermediates,” that are the proximate cause of adverse effects in the cell. Reactive intermediates are so named because they are chemically unstable and tend to chemically modify or interact with critical molecules in the cell. These critical molecules include DNA, protein, and lipids. Nephrotoxicants or reactive intermediates derived from their metabolism may also generate partially reduced forms of molecular oxygen (so-called reactive oxygen species (ROS)). ROS are, as the name implies, similar to reactive intermediates in being chemically unstable with a consequent tendency to modify or interact with critical cellular molecules. Similar to the discussion in the previous paragraphs, these interactions may lead to a range of cellular responses, including cell repair, cell death, or mutations that can ultimately result in genetic transformation of the renal cell. Figure 7.5 Scheme of biochemical mechanisms by which nephrotoxic chemicals affect renal cells. For the most part, chemically induced nephrotoxicity is dependent on metabolism or biotransformation to generate a reactive intermediate. Such reactive intermediates may form covalent adducts with cellular DNA, lipid, or protein, thereby altering function, or they may promote or generate reactive oxygen species (ROS). ROS may in turn cause oxidative damage to critical macromolecules, including DNA, leading to altered gene expression and genetic transformation, or may lead to cytotoxicity. As shown in Figure 7.4, the cell injury may lead to cell death or promotion of repair, depending on the extent of the injury and the energy and redox status of the cell. One’s ultimate goal in treating patients who have been exposed to nephrotoxic chemicals is to monitor kidney function by measurement of various parameters in either urine or blood. These so-called biomarkers are also used in many in vivo animal studies to monitor kidney function in an intact organism. Biomarkers can be used for different purposes. For example, parameters that change in response to alterations in kidney function are called biomarkers of effect. These are important in quantifying the consequences of toxicant exposures. Besides treatment, we are also interested in prevention of kidney injury. Hence, changes in parameters that occur very quickly after an exposure has occurred but prior to the onset of renal functional changes are termed biomarkers of exposure. One of the primary means of assessing renal function in the whole animal or in patients is to monitor GFR. GFR is defined as the volume of blood completely removed of a substance per unit time (ml/min); GFR = 125 ml/min in healthy, young humans. Two commonly used indicators of GFR are blood urea nitrogen (BUN) or serum creatinine (SCr) levels. Nitrogenous wastes derive from protein and amino acid degradation. Under normal conditions, amino acids are reabsorbed by the proximal tubules and breakdown products of proteins are efficiently excreted, with much of the N-containing waste products being excreted as ammonia or ammonium salts. Under conditions of impaired renal function, however, the efficiency of nitrogenous waste excretion is diminished, leading to increased retention of N-containing compounds. Creatinine (Mr = 113) is released from skeletal muscle due to the nonenzymatic hydrolysis of creatine. The rate of creatinine production is generally constant and proportional to muscle mass. In a normal, healthy state, glomerular filtration is nearly complete so that very low levels of creatinine remain in the blood. Using creatinine clearance (C-Cr) as an index of renal function, GFR is calculated by the following equation:
NEPHROTOXICITY: TOXIC RESPONSES OF THE KIDNEY
7.1 RENAL STRUCTURE AND PHYSIOLOGY
Overview of Kidney Structure and Function
Filtered (mEq/24 h)
Excreted (mEq/2 h)
Reabsorbed (mEq/24 h)
Reabsorbed (%)
Sodium
23,900
100
23,800
99.6
Chloride
19,800
100
19,700
99.5
Bicarbonate
5,100
2
5,098
99.9
Potassium
680
50
630
92.6
l/24 h
l/24 h
l/24 h
Water
169
167.5
1.5
99.1
Nephron Structure and Heterogeneity
Property
Proximal tubule (PT)
Distal convoluted tubule (DCT)
Medullary thick ascending limb (mTAL)
Shape
Large, cuboidal
Small, cuboidal
Flattened
Intrarenal localization
Cortex/OSOM
Cortex
OM
Membrane structure
High-density BB microvilli, extensive BL invaginations
Few BB microvilli, few BL invaginations
Few BB microvilli, few BL invaginations
Mitochondrial density
High
Moderate
Moderate
Oxygenation
High
High
Low
Energetics
High OXPHOS, FA oxidation, gluconeogenesis
Glycolysis, low OXPHOS
Glycolysis, low OXPHOS
Transport function
OA–, OC+, glucose, amino acids; water-permeable
Na+, K+, H+, HCO3–; water-permeable
Divalent cations, Na+–K+–Cl–; low water permeability
Drug metabolism
CYP, FMO, GSH-dependent
Low
PGS
Countercurrent Flow and Urinary Concentration Mechanism
Membrane Transport Processes
Summary of Physiological Factors Contributing to the Kidneys as a Target Organ for Toxic Chemicals
Parameter
Comments
1. Blood flow
The two kidneys comprise <1% of total body weight but receive 20–25% of cardiac output.
2. Concentrating mechanisms
Countercurrent renal blood flow minimizes loss of solutes from tubule or lumen and leads to intracellular accumulation.
3. Filtration
Glomerular filtration: Ultrafiltrate of plasma; 125 ml/min in healthy, young adults.
4. Absorption and secretion
Absorption: Lumen to cell to blood; Secretion: Blood to cell to lumen; plasma membrane transporters for organic anions and cations, inorganic ions, metabolites; active transport
5. Bioactivation
Drug metabolism enzymes generate intracellular reactive metabolites.
7.2 CLASSIFICATIONS OF RENAL INJURY
Acute Kidney Injury
Chronic Kidney Injury
Responses at the Cellular Level
Factor (1)
Factor (2)
Factor (3)
Factor (4)
7.3 ASSESSMENT OF RENAL FUNCTION AND INJURY IN THE CLINIC AND IN IN VIVO ANIMAL MODELS
Measurement of GFR
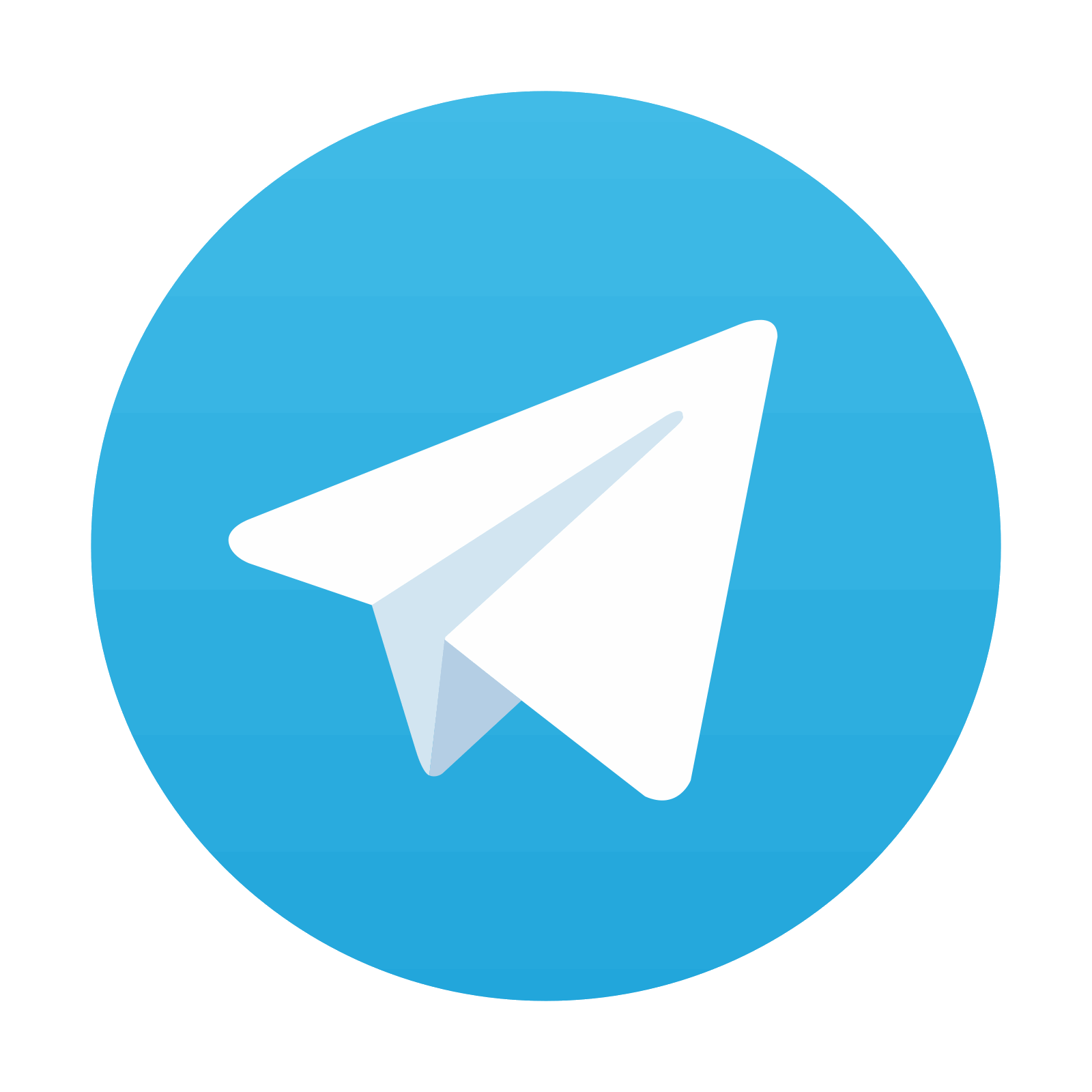
Stay updated, free articles. Join our Telegram channel
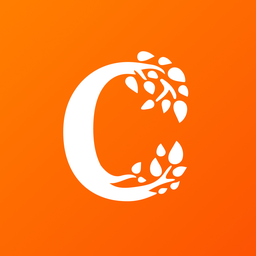
Full access? Get Clinical Tree
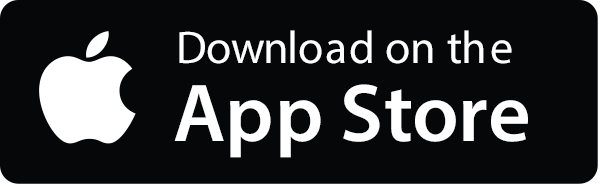
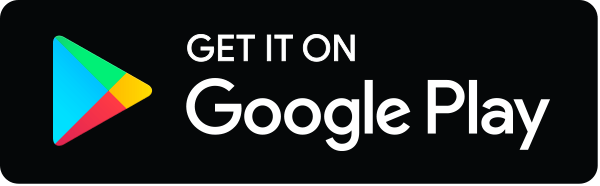