19 Hongbo Ma, Steve Diamond, Georgia Hinkley, and Stephen M. Roberts Nanotechnology represents one of the most promising technologies of the twenty-first century and has been considered to be a new industrial revolution. It is defined as the development and application of materials and structures with nanoscale dimensions, usually in the range 1–100 nm. The prefix “nano” is derived from the Greek word “nanos” meaning “dwarf.” Nanomaterials refer to any materials with at least one dimension in the range of 1–100 nm (1 nm = 10−9 nm). Particles in the nanosized range have been present on earth for millions of years. Soot, for instance, a product of incomplete combustion of fossil fuels and vegetation, has a particle size in the nano- to micrometer range and therefore falls partially within the “nanoparticle” domain. Only recently nanoparticles have attracted great attention because of the increasing manufacturing and manipulation of such materials by humankind. Therefore, the term “nanoparticles” usually applies only to engineered particles but not particles under 100 nm that occur naturally or are by-products of other processes such as welding fumes, fire smoke, or carbon black. Nanomaterials often display novel properties as compared to their bulk counterparts, such as enhanced magnetic, catalytic, optical, electrical, and mechanical properties. For example, inert elements like platinum or gold become excellent chemical catalysts when in nanoscale. Normally stable aluminum becomes combustible and copper becomes transparent. Such novel properties of nanomaterials have rendered their increasing application in a broad range of areas, from industry products such as tires, catalysts, electronic component, window sprays, paints, and coatings to commercial products such as sporting goods and personal care products and medical applications such as antimicrobial agents, clinical diagnosis, imaging, and drug delivery. A report by the U.S. National Science Foundation in 2006 estimated that the global market for nanotechnology may reach $1 trillion or more within 20 years. The novel properties of nanomaterials, and the challenges in evaluating their toxicity, have led to the development of nanotoxicology as a new field. This chapter will discuss: Nanomaterials are broadly defined by sizes. In theory, all conventional materials like metals, semiconductors, glass, ceramic, or polymers can be engineered with a nanoscale dimension. Therefore, there are different approaches for classification of nanomaterials. The most widely accepted classification is based on chemical composition, from which nanomaterials can be classified into four main categories: carbon-based nanomaterials, metal/metal oxide nanomaterials, quantum dots (QDs), and dendrimers. Carbon-based nanomaterials include fullerene, carbon nanotubes (CNTs), and related products. Manufacturing of all these nanomaterials originates from the discovery in 1985 of fullerenes (also known as buckyballs), a 60-carbon atom hollow sphere. Fullerenes and CNTs are produced in quantities as high as 1500 t/year by companies such as Frontier Carbon Corporation in Tokyo, Japan, and Fullerene International Corporation in Tucson, AZ, United States. Due to their excellent thermal and electrical conductivity, these materials are widely used in plastics, catalysts, battery and fuel cell electrodes, sensors, water purification systems, and automotive industries. Several varieties of organic nanoparticles have been engineered by the pharmaceutical industry for drug delivery, providing a variety of advantages compared to conventional systems. Nanoparticle drug delivery is particularly advantageous for acid-sensitive oral medications such as protein or RNA-based therapies. These systems provide safe passage through the harsh gastric environment and deliver therapeutics to absorptive surfaces in the small intestine, often designed to degrade in a neutral pH solution. Other routes of administration with certain applications can also greatly benefit from using nanoformulated delivery systems. For example, the harsh side effects of chemotherapy drugs can be lessened by nanodelivery systems that are coupled to a targeting agent designed to release medications at the site of a tumor. Liposomes, solid lipid nanoparticles, polymeric micelles, mesoporous silica particles, and nanocrystals are just a few examples of engineered nanoparticles used in the pharmaceutical industry. Metal-containing nanomaterials have received considerable attention and have a broad range of applications. Titanium dioxide (TiO2) and zinc oxide (ZnO) are widely exploited for their photocatalytic properties in industrial applications such as solar cells, paints, and coatings. They are also finding extensive applications in sunscreens and cosmetics due to their excellent UV-blocking capability and visible transparency at nanoscale. Production of these metal oxides for use in skincare products is estimated to be 1000 t/year in 2005–2010. Cerium dioxide (CeO2) is mainly used as a combustion catalyst in diesel fuels to improve combustion efficiency and control emissions. The most widely used nanoparticulate zerovalent metals include silver (Ag), iron (Fe), and gold (Au). Nanosilver has been incorporated into the greatest number of consumer product applications due to its excellent antimicrobial and antiviral activities. Nanoscale iron is most widely used in environment remediation of waters, sediments, and soils. Nanoscale gold has been used in medical applications as vectors in tumor therapy. QDs are semiconductor nanocrystals that have extraordinary optical and electrical properties due to the quantum-confined nature of their energy levels. They are usually composed of a reactive core (such as cadmium selenide [CdSe], cadmium telluride [CdTe], or zinc selenide [ZnSe]) that controls its optical properties and a protective shell (such as silica or ZnS) that protects the core from oxidation and enhances the photoluminescence yield. QDs are used largely in medical applications such as diagnostic imaging or targeted therapeutics. Extended applications of these QDs include solar cells and photovoltaics, security inks, photonics, and telecommunications. Dendrimers are multifunctional polymers whose size, topology, flexibility, and molecular weight can be controlled. Their applications typically involve conjugating other chemical species to their surface, which can function as detecting agents, affinity ligands, targeting components, imaging agents, or pharmaceutically active compounds. Nanomaterials are also being used as direct food additives and in food packaging materials as antibacterial agents. Nanosized silica and titanium dioxide are both used in a number of food products—silica as an anticaking and thickening agent for dry food products and titanium dioxide as a whitening agent in many candies and gums. Sources estimate that the current human exposure to nanosized silica and titanium dioxide from food exceeds 40 mg per person per day. In addition, silver and zinc nanoparticles are being used in food packaging, for example, absorbent meat pads, as antibacterial agents to extend the shelf life of foods. Release of embedded particles from packaging materials may lead to further human exposure to nanomaterials from foods. With increasing use in commercial and consumer products, both intentional and unintentional exposures to nanomaterials and their release into the environment are inevitable. Unintentional release includes atmospheric emissions and solid or liquid waste discharge during manufacturing processes and intentional release includes their uses in remediation of environmental contamination, as well as in consumer products such as paints, fabrics, and personal care products. In some cases, disposed nanoparticles will ultimately be deposited on land and surface water systems and have the potential to interact with biota. Atmospheric emissions (e.g., diesel fumes) may lead to inhalation exposure in humans and animals, and any waterborne contamination is also of concern for dermal and ingestion exposure. Certain synthetic nanoparticles have been detected in the environment, and modeled concentration of engineered nanomaterials can reach μg/l levels (Table 19.1). The very same properties that lead to the technical advancement in nanotechnology may also lead to unexpected and unanticipated consequences upon interaction with biological systems. For example, the small sizes of nanomaterials impart a different biokinetic behavior of the materials and enable them to reach more distal regions of the body compared to their bulk counterparts. To fully recognize the benefits of nanotechnology and to ensure a sustainable development and application of it, it is essential to understand its implications on human and environmental health at an early stage. Concerns have been raised from both scientific community and general public regarding the environmental health and safety of nanotechnology. Table 19.1 Predicted Environmental Concentrations of Engineered Nanomaterials (ENMs) in the Environment in Europe and the United States Numbers in parentheses are the lower and upper quantiles (Q0.15 and Q0.85). For surface and sewage treatment plant (STP) effluents, the numbers represent 2008 ENM concentrations; for sediment and soil, the numbers illustrate the annual increase of ENM concentrations. Nanotoxicology is the study of toxic effects of nanomaterials. It is proposed as a new branch of toxicology to address the gaps in knowledge concerning the toxicological effects caused by nanomaterials. It encompasses the physicochemical properties of nanomaterials, routes of exposure, toxicokinetics and toxicodynamics, and regulatory aspects. It also involves proposing and developing reliable and robust test protocols for nanomaterials in human and environmental risk assessment. It should be pointed out that naturally occurring nanoparticles, such as nanosized metal oxides, exist in all ecosystems and the living organisms have adapted to them during evolution. However, the potential harmful properties of synthetic nanomaterials have to be evaluated as toxicological data on these materials are just emerging. Nanoscale materials behave differently than their macroscale counterparts because of their smaller size. Below about 100 nm, the behavior of elements follows the rules of quantum physics. Gravity is not important and van der Waals forces govern the attraction/repulsion behavior between particles. Surface charge becomes very important and agglomeration of particles occurs readily, depending on environmental conditions. Some nanoparticles can easily penetrate cell walls and membranes without the requirement of phagocytosis for entering cells. Toxicity or behaviors that are surface related are exacerbated by the increasing surface-to-volume ratios at the nanoscale. These novel and unique properties of engineered nanomaterials bring special challenges to nanotoxicology as compared to conventional toxicology studies. Characterization of the physicochemical properties of nanomaterials is critical because these are usually the determinants of biological activity and effects of the nanomaterials. Characterization of nanomaterials is much more complex and indispensable as compared to their larger counterparts (which are usually confined to chemical composition and purity determination), because nanomaterials can differ widely in terms of particle size, shape, surface area, surface chemistry, crystallinity, solubility, agglomeration state, and chemical composition. There has been a consensus within the scientific community that thorough characterization of nanomaterials is essential during toxicological studies, although disagreement exists regarding the key “essential” characterization information to be obtained. These properties may include, but are not limited to, aggregation/agglomeration, size, dissolution (solubility), surface area, surface charge, and chemical composition. In addition, some key abiotic factors in the aqueous media, such as pH, presence of natural organic matter (NOM), and ionic strength, should also be measured. In practice, complete characterization is not always possible due to extensive instrumentation and specialized technical expertise required; therefore, the extent of characterization usually depends on objectives of a specific study and prioritizing characterization measurements on the basis of their known or suspected influence on toxicity. A variety of methods are available for characterizing nanoparticles. These include high-resolution imaging techniques such as scanning and transmission electron microscopy (SEM/TEM) that can be used to measure the shape, size, and crystalline nature of the particles. Size is usually the most important criterion that differentiates a nanoparticle for functionality among all attributes of the nanomaterial. Toxicity data is meaningless without proper determination of size and/or size distribution of the test material. Nanoparticles tend to agglomerate in environmental or biological fluids. Agglomeration involves adhesion of particles to each other mainly due to van der Waal’s forces that become quite prominent at nanoscale due to increased surface area-to-volume ratios. The rate and extent of agglomeration may vary based on interaction with macromolecules and biological fluids once the nanoparticles are introduced inside living systems. A nanoparticle suspension is a dynamic system as agglomeration or dissolution is ongoing and agglomeration is highly dependent on physiochemistry of the solution. Agglomeration behavior is expected to affect nanotoxicity as it changes the size, surface area, and sedimentation properties of the nanoparticles and should be considered for toxicity studies. The most commonly employed techniques for particle size determination are Brunauer–Emmett–Teller (BET), dynamic light scattering (DLS), and TEM, although a variety of methods are available. The BET method is typically used for the calculation of surface area of solids by physical adsorption of gas molecules on the solid surface. TEM gives direct measurement of particle size, size distribution, and morphology by image analysis. DLS has the ability to provide the size and size distribution information in relevant liquid media used for exposure. Each technique has its own advantages and disadvantages and no single technique is appropriate for all materials. Techniques for nanoparticle surface analysis include electron spectroscopies, ion-based methods and low-energy ion scattering, and scanning probe microscopy (including atomic force microscopy and scanning probe microscopy). Solubility of nanoparticles can be measured by ultrafiltration, dialysis, and flow field fractionation coupled to inductively coupled plasma mass spectrometry (ICP-MS). Adequate characterization of nanoparticles often involves a variety of complementary analysis methods. However, none of these methods are well suited to quantifying the amount of nanoparticles in biological (tissues and fluids such as blood) or environmental media (soil, sediment, or water), which remains an area of high research need. Another unique challenge facing nanotoxicology is that there are large numbers of materials to be tested and the numbers keep increasing as new materials are developed and produced. As it is not feasible to conduct toxicity test for every nanomaterial, it is highly desirable for the scientific community to have access to well-characterized nanomaterials with known toxicity and preferably a well-documented toxicity mechanism that may be used as reference materials for hazard assessment. Reference materials play an important role in particle toxicology as particles may have variable toxicity depending on type, origin, chemistry, size distribution, etc. Reference materials have been used historically in particle toxicity studies. For example, asbestos fibers from five different locations in the world and with specific physicochemical properties are prepared by the Union of International Cancer Control as reference materials for toxicity study of asbestos. Development of reference materials for nanomaterial toxicity testing is just beginning. A set of nanoparticle reference standards consisting of colloidal gold with nominal diameters of 10, 30, and 60 nm was released by National Cancer Institute in 2007. A number of international organizations and initiatives are underway to develop reference materials for nanotoxicology studies. For instance, the Organisation for Economic Co-operation and Development (OECD) has produced a list that comprises fullerenes, single- and multiwalled CNTs, carbon black, polystyrene, dendrimers, nanoclays, and nanoparticles of Ag, Fe, TiO2, Al2O3, CeO2, ZnO, and SiO2. All these materials are already in commercial production and applications. Other common difficulties with test materials include consistency of the materials and quantities available for study. As manufacturing processes for new nanomaterials are being worked out, the nanomaterials can vary substantially from lot to lot with respect to key characteristics. This reinforces the need to characterize each batch or lot of test material received to insure that it meets the intended specifications. Also, new nanomaterials may be available only in very small quantities (e.g., milligram amounts). This can make obtaining quantities necessary to perform toxicity studies, especially in vivo studies, difficult. Accurate assessment of toxicity relies on correct assessment of dose–response relationships. The dose metric is one of the critical factors that determine the shape of dose–response curves. In general, toxicologists express doses by mass. However, this may not be the best approach for nanomaterials given their extreme low mass and high surface area-to-volume ratio. Size and surface area are two important properties from a toxicological perspective. As size decreases, the proportion of surface atoms increases making the particle surface more reactive. Such enhancement of surface reactivity at nanoscale is usually responsible for the nanoparticle-elicited biological response. Therefore, it is generally believed that particle surface area is a more appropriate dose metric than the traditional mass-based measure of dose. For example, particle BET surface area has been suggested as the most appropriate parameter to evaluate the inflammatory potential of ultrafine carbon particles in mice bronchoalveolar lavage. Similar conclusions have been reached for titanium dioxide nanoparticles in terms of their capacity for reactive oxygen species (ROS) generation. This is consistent with the mechanistic consideration that cellular response may be related to surface area of a nanoparticle due to effects arising from particle surface chemistry/reactivity. Particle number represents another candidate dose metric. This is especially true when the toxic responses of nanoparticles are a result of obstruction of cellular processes by the physical presence of poorly soluble particles. The number of particles in the exposure medium has been considered to be the best parameter to describe the toxicological effects of different types of nanoparticles on acute lung inflammation in rats and mice after a comparison of four types of dose metrics: particle number, joint length (product of particle number and mean size), BET surface area, and calculated particle size based on surface area. The complexity of particle properties and mechanisms of action preclude generalization of the appropriate dosimetry for all nanoparticles. Surface area, particle number, or even mass per volume could be the relevant dose metric in risk assessment of a given material, depending on the specific test conditions and the biological effects of interest. As the variability in results of similar nanotoxicity studies will continue to exist, it is necessary to analyze the results obtained with respect to different types of dose metrics so that a convincing conclusion regarding the comparative toxicity of a nanomaterial can be reached. This in turn will help in deciding the suitability of different dose metrics for nanotoxicological studies. It should be noted that even selection of a suitable dose metric for in vitro nanotoxicity test is not enough for deciding cellular dose without considering other factors associated with nanoparticles. Unlike soluble chemicals, particles can diffuse, agglomerate, and settle depending on their physicochemical properties and media characteristics. Therefore, the dose introduced to the exposure system does not necessarily reflect the dose reaching the biological target. Yet in most in vitro nanotoxicity studies, it is the administered dose or media concentration that is measured as done in classical toxicology. In addition to considering dosimetry issues when designing a nanotoxicology study, it is important to evaluate the appropriateness of toxicity assays for the materials being tested. Standard toxicity assays were designed and validated for soluble chemical materials. Particulate matter presents a unique situation because these materials may possess physiochemical properties that are able to interfere with standard assays. Due to their high surface area-to-volume ratio, particles are capable of adsorbing large quantities of proteins and many particle types also have surface catalytic properties. Both of these attributes may cause assay interference. In addition to direct interaction with assay components, particles have the potential to interfere with endpoint measurement through spectral properties. Metallic particles have significant potential for interference via light absorption and fluorescent particles via light emission and/or absorption. It is important to consider and control for the potential of assay interference when performing standard toxicity assays with nanomaterials. Human exposure to nanomaterials is likely to occur incidentally as well as intentionally via several routes including inhalation, ingestion, and dermal exposure. Inhalation exposure to particulate matter has been studied for quite some time and is most well understood. The most important aspect for inhalation bioavailability is particle size—particles larger than 10 μm are trapped in the nose and upper airways, while particles less than 10 μm are able to deposit much lower in the respiratory tract in a size-dependent manner. Particles with a diameter less than 250 nm are able to translocate into the bloodstream and achieve systemic circulation. Similarly to inhalation exposure, ingestion of nanomaterials leading to systemic exposure is also largely size dependent. Particles greater than 3 μm are generally not well absorbed in the gastrointestinal tract (GIT), while particles near 1 μm in diameter are available for immune-mediated uptake via specialized intestinal immune centers called Peyer’s patches. Smaller particles can achieve uptake via enterocytes, either passively through cell turnover or actively through receptor-mediated uptake. However, it should be noted that overall oral bioavailability of most particle types is quite low. Dermal absorption of intact particles through noncompromised skin is almost nonexistent. For all routes of exposure, there are two very important caveats to consider that can greatly alter bioavailability: dissolution and agglomeration. As size is an important aspect for systemic uptake following all three routes of exposure, processes that affect particle size are always important to evaluate. Dissolution in the pulmonary and gastric environments can greatly increase overall bioavailability, by both decreasing average particle size and through the uptake of ions. Dissolution can also greatly increase dermal bioavailability; however, dermal exposure presents a special situation due to the potential for UV interaction with deposited particles. Breakdown of particles by UV exposure can even further increase dermal bioavailability. In contrast to dissolution, agglomeration in high-salt biological environments will have the opposite effect for all routes of exposure, resulting in decreased bioavailability. In addition to particle properties that are likely to influence bioavailability, any compromise to the barrier integrity of the GIT or skin is likely to increase bioavailability and should be considered. As mentioned earlier, the toxicology of inhaled particulate matter has been studied for centuries but has more recently been expanded to include a category for nanosized or “ultrafine” particles. Inhaled particles that achieve alveolar deposition have the potential to cause inflammation, leading to several diseases including asthma, fibrosis, chronic obstructive pulmonary disease, and cancer. Epidemiological analysis of occupational exposure to various metal and organic dusts has been shown to have an association with the development of fibrosis and cancer; however, these associations are confounded by exposure to a mixture of particles from a wide range of sizes. Rodent studies have shown that subchronic and chronic exposure to silver, titanium dioxide, zinc oxide, and copper oxide particles causes an inflammatory response in the lung. Inhalation exposure to nanosized fibers like CNTs has been shown to cause fibrotic-like responses, similar to those observed in asbestos-exposed humans. In general, much less toxicity is observed for ingested nanomaterials. The GIT tissue is protected from direct toxicity by a thick mucin layer, and systemic toxicity is limited due to low overall bioavailability. Subacute exposure to zinc oxide in rats has been shown to cause changes in clotting times and dose-dependent increases in aspartate aminotransferase (AST) and alanine aminotransferase (ALT) levels, and subchronic exposure to silver in rats causes dose-dependent increases in alkaline phosphatase (ALP). In addition to direct tissue and systemic toxicity, disruption of GIT microbiota is a potential toxic response to ingestion of nanomaterials due to the antibacterial properties of many metal particles. Other than the case of beryllium, systemic toxicity due to dermal nanoparticle exposure is quite rare. Due to the known reactions of the bulk materials, metal particles such as nickel and cobalt have the potential to cause allergic-type reactions, such as contact dermatitis. Beryllium presents a special case in which a lung disorder known as chronic beryllium disease can be initiated by dermal exposure to beryllium dust. This disorder requires both a sensitization phase, achieved by inhalation or dermal exposure to beryllium, followed by a progression phase of beryllium inhalation. Like most occupational exposures, these associations are confounded by exposure to a large range of particle sizes, but it can be expected that a portion of the dust generated during beryllium processing is in the nanorange. When considering the observed toxicity of nanomaterials, it is important to consider the role of dissolution, particularly for metal particle exposures. Many metals in the ionic form are known to be toxic (e.g., copper) and may account for a portion or all of observed toxicity. Especially following ingestion, when particles can be exposed to the harsh pH conditions of the stomach for more than 2 h, dissolution should be carefully considered and measured when possible. Nanomaterials may be released to the air, soil, or water both unintentionally and intentionally. Nanoparticles can be highly mobile in the environment due to their small size and light mass. Once entering into the environment, nanomaterials undergo abiotic interactions with a variety of environmental factors, leading to physical and chemical alternations of the materials. These alternations usually determine the transport and fate of nanomaterials and consequently their bioavailability and toxicity to environmental receptors. A logical chain of events leading to nanomaterial toxicity in the ecosystem is shown in Figure 19.1. Figure 19.1 The logical chain of events accounting for the bioavailability and toxicity of nanomaterials starts with the nanomaterials’ entrance into the ecosystem, proceeds by abiotic interactions between the nanomaterials and environmental factors, leads to bioavailability and toxicity to ecological receptors, and finally results in effects on population, community, and ecosystem levels. Source: Reprinted with permission from Navarro et al. (2008). © 2008 Springer.
NANOTOXICOLOGY
19.1 CLASSIFICATION OF NANOMATERIALS AND THEIR POTENTIAL APPLICATIONS
Surface Water (µg/l)
STP Effluent (µg/l)
Sediment (Δμg/kg/year)
Soil (Δμg/kg/year)
TiO2
Europe
0.015 (0.012, 0.057)
3.47 (2.50, 10.80)
358 (273, 1409)
1.28 (1.01, 4.45)
United States
0.002 (0.002, 0.010)
1.75 (1.37, 6.70)
53 (44, 251)
0.53 (0.43, 2.13)
ZnO
Europe
0.010 (0.008, 0.055)
0.432 (0.340, 1.42)
2.90 (2.65, 51.7)
0.093 (0.085, 0.661)
United States
0.001 (0.001, 0.003)
0.3 (0.22, 0.74)
0.51 (0.49, 8.36)
0.050 (0.041, 0.274)
CNT
Europe
0.004 (0.003, 0.021)
14.8 (11.4, 31.5)
241 (215, 1321)
1.51 (1.07, 3.22)
United States
0.001 (0.001, 0.004)
8.6 (6.6, 18.4)
46 (40, 229)
0.56 (0.43, 1.34)
Ag
Europe
0.764 (0.588, 2.16)
42.5 (32.9, 111)
952 (978, 8593)
22.7 (17.4, 58.7)
United States
0.116 (0.088, 0.428)
21.0 (16.4, 74.7)
195 (153, 1638)
8.3 (6.6, 29.8)
Fullerenes
Europe
0.017 (0.015, 0.12)
5.2 (4.23, 26.4)
17.1 (6.22, 530)
0.058 (0.057, 0.605)
United States
0.003 (0.002, 0.021)
4.6 (4.49, 32.66)
2.5 (1.05, 91.3)
0.024 (0.024, 0.292)
19.2 HOW IS NANOTOXICOLOGY DIFFERENT?
Importance of Characterization
Issues with Test Materials
Issues with Dosimetry
Suitability of Standard Toxicity Tests
19.3 HUMAN NANOTOXICOLOGY
Bioavailability of Nanomaterials
Toxicity of Nanomaterials
19.4 ENVIRONMENTAL NANOTOXICOLOGY
Environmental Fate and Transport of Nanomaterials
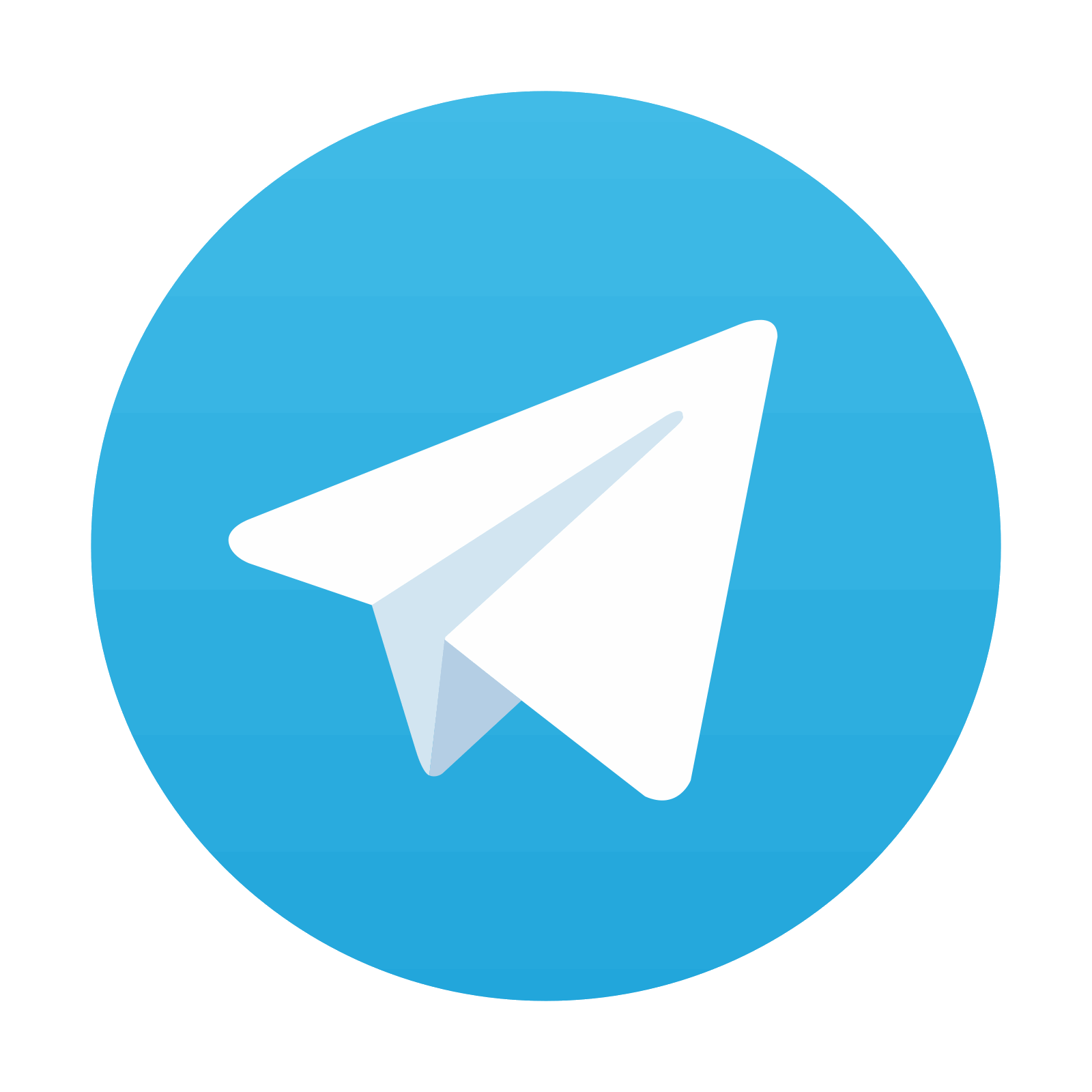
Stay updated, free articles. Join our Telegram channel
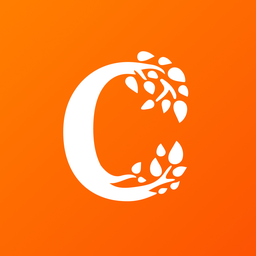
Full access? Get Clinical Tree
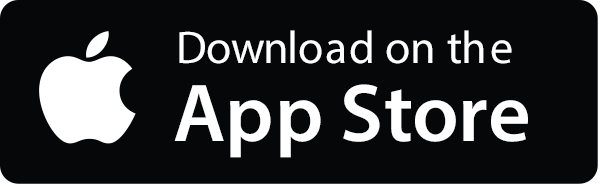
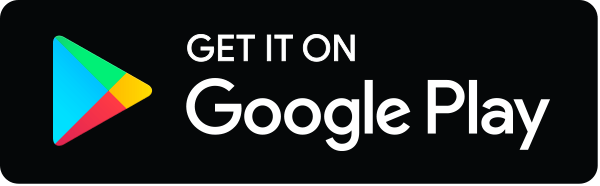