14 Martha M. Moore, Meagan B. Myers, and Robert H. Heflich Genetic toxicology is the subfield of toxicology that deals with damage to the genetic material. It includes the study of mutations, alterations in chromosome number, damage to chromosomes and primary DNA damage that may or may not ultimately lead to a heritable mutation. Genetic toxicology is a part of applied toxicology. Genetic toxicology data are used in product development, particularly for various hazard assessments, and are either required for, or often available for, use in a number of different types of regulatory decisions. In general, these regulatory decisions can be divided into hazard identification and mode-of-action assessment for chemicals inducing either cancer or mutation. A large variety of products (e.g., pharmaceuticals, pesticides, new use chemicals, food additives, and food contact substances) are tested for their potential to induce genetic damage. The two major human health outcomes of concern are cancer and impaired reproduction (Figure 14.1). Figure 14.1 Mutations can occur in somatic cells or in germ cells and cause a wide variety of adverse health outcomes. Gene mutations and chromosomal mutations (both structural and numerical), along with cellular toxicities, epigenetic effects, and alterations of gene expression, are all involved in the development of cancer. Germ cell and related tissue mutations can lead to decreased fertility, increased spontaneous abortions and other birth defects, or to new heritable mutations that are passed on to future generations. While it has not been shown that human germ cell mutations can be induced by chemicals and passed on to future generations, it is clear that these effects occur in laboratory animals and it is also clear that new mutations have occurred in human families. This chapter will cover several aspects of genetic toxicology with a focus on its regulatory use and will be organized as follows: Deoxyribonucleic acid (DNA) is the fundamental structure that contains all of the information required for cellular function and the information encoded in DNA is passed from one cell generation to the next. DNA is comprised of two antiparallel strands of nucleotides that are linked together to form a double helix. These two strands are held together by hydrogen bonds that form between the complementary nucleotide bases (Figure 14.2). There are four nucleotide bases in DNA (Figure 14.3), two purines (guanine (G) and adenine (A)) and two pyrimidines (cytosine (C) and thymine (T)). To form the double helix, a purine pairs with a pyrimidine. Specifically, A pairs with T and G pairs with C. The ordering of the DNA bases (i.e., the DNA sequence) ultimately controls the function of the molecules that make up cells. The DNA is transcribed into RNA (ribonucleic acid) where uracil is used instead of thymine. RNA serves as the template upon which a series of amino acids are assembled to make a protein. The sequence of amino acids is determined by the order of the nucleotide bases that are decoded in groups of three bases called codons. Each codon indicates a specific amino acid and the order of the inclusion of amino acids into the protein. Figure 14.2 The DNA double helix is comprised of four different nucleotides that form base pairs held together by hydrogen bonds. Figure 14.3 The purine and pyrimidine bases, phosphate group, and pentose sugars comprise DNA or RNA. When a cell divides, the two strands of DNA separate from each other and, using one of the DNA strands as a template, the cellular machinery replicates the strand. When this occurs without error, the daughter cells contain the same DNA sequence as their parent cell. Errors can occur in this replication process, however, and the order of bases can be modified, thus resulting in a mutation (an alteration in the DNA nucleotide sequence order). These types of mutations are “spontaneous” mutations, that is, they occur as a result of the normal process of cell replication and division. Mutations also can occur following exposure to chemicals or physical agents such as radiation. The DNA is packaged along with proteins into an organized structure called the chromosome. Each chromosome contains a single piece of DNA that includes a large number of genes, regulatory elements, and other nontranscribed nucleotide sequences. Chromosomes are microscopically visible structures and each species has a defined number. Because chromosomes occur in pairs, each cell will contain two copies of each gene, with the exception of the sex determining chromosomes where, for humans (and other mammals), women have two X chromosomes but men have one X and one Y chromosome. These gene copies may not be exactly identical. There can be small variations in the DNA sequence of an individual gene that are not lethal to the cell or organism but that may cause alterations in cellular function. There are a number of human diseases (discussed below) that result from small alterations in genes. These slightly different genes are called alleles. If a particular gene has two identical copies on the two chromosomes, this is designated as homozygous. If the two alleles are different, the cell is heterozygous. Mutations can be classified as point mutations, gene mutations, or chromosomal mutations; the primary distinction between the various types relates to the number of base pairs altered. Point mutations impact a single base pair and can be base pair substitutions or frame shifts (Figure 14.4). If a purine is exchanged for a purine or a pyrimidine for another pyrimidine, it is designated as a transition. On the other hand, if a pyrimidine replaces a purine or vice versa, it is called a transversion. Base pair substitutions that occur in the coding regions (the part of DNA responsible for ultimately producing a new protein), can be further classified based on their effect on the protein product. A silent mutation occurs when the base pair change does not impact the amino acid order in the protein. This can occur because more than one codon can code for a particular amino acid. A missense mutation occurs when the altered codon designates an incorrect amino acid into the protein and a nonsense mutation codes for a stop codon. In the latter case, the protein will be truncated and will generally not be functional. The three base pair codons consisting of UAA, UGA, or UAG are stop codons, which indicates to the cellular machinery that the protein is complete and no further amino acids should be added. Frame shift mutations involve the insertion or deletion of a small number of base pairs, which impacts the reading frame. That is, it will dramatically affect the three base or codon order, generating a protein containing an abnormal order of amino acids, which generally results in a premature stop codon at some point in the sequence. Figure 14.4 Single base changes can alter the normal DNA sequence and result in either a base pair substitution mutation or a frame shift mutation. Gene mutations or chromosomal mutations can contain intragenic insertions or deletions. The size of the insertion or deletion will determine whether the impact is to a single gene, a series of linked genes, or a whole chromosome. An intragenic deletion may cause the loss of several introns and exons from a single gene. These deletions may lead to heterozygosity for homozygous alleles or to loss of heterozygosity (LOH) which is the loss of one allele of a heterozygous pair of alleles. A very large deletion can result in the loss of multiple genes. In addition to insertions and deletions, there are several types of chromosomal rearrangements. Gene amplifications, translocations between chromosomes, inversions of chromosome sections, and mitotic recombination and gene conversion can all lead to rearrangements of the chromosomes and therefore a rearrangement of genes or parts of genes. If these events are large enough, they can be visualized under a microscope by someone trained in evaluating gross chromosome aberrations. Mutations can occur spontaneously, for instance, by errors in DNA replication and DNA repair. Every organism has a spontaneous mutation rate, defined as the number of mutations per locus that are incurred either per cell division or, at the organism level, per generation. This rate of spontaneous mutation can vary across species, with some bacteriophage having rates as low as 1 in 109 loci. Higher organisms such as corn, Drosophila, and humans have rates of approximately 1 in 105–107 loci. In general, higher animals and plants have genes that spontaneously mutate at a much higher rate than those in microorganisms. It is unclear why this should be the case, but perhaps the basic difference is between diploid and haploid organisms. The former has no normal allele to offset the deleterious effects of a new mutation, while the latter are able to survive as long as a single normal gene can provide sufficient normal protein for cell survival. That is, new spontaneous mutations can be recessive in function if the cell is diploid, but in haploid cells they are effectively dominant and also generally lethal. It should be noted that in rare instances some new mutations may provide the organism a selective advantage and this is the basis for evolutionary modifications. Since the demonstration by H. J. Muller in the early twentieth century, that X-rays can induce new mutations, the science of studying the ability of chemical and physical agents to cause genetic damage has grown rapidly. The field of genetic toxicology took shape in the 1960s and 1970s when scientists developed methods to assess mutation in a quantitative way using a wide variety of organisms from bacteria, yeast, Drosophila, Neurospora, plants, mammalian cells and various rodent species as model systems. Penetrating ionizing radiation, including X-rays, gamma rays from radium and other radioactive atoms, and neutrons from nuclear reactors, has been widely studied. The first chemicals to be identified as mutagens include mustard gas, and various nitrogen or sulfur–mustard compounds. A chemical that causes chromosome breakage is a clastogen. Clastogens are generally defined as those chemicals that can cause chromosome damage that can be seen using a microscope. If there is damage to the cellular machinery involved in cell division and chromosome pairs do not all separate normally into the two daughter cells, this nondisjunction will result in aneuploidy, an abnormal number of chromosomes in the daughter cells. Polyploidy results when there are full (or almost full) additional sets of a chromosome complement. Following the metabolism of a chemical and its transport through the body and cells to reach the genetic material in the cell nucleus, there are a number of biological events that can lead to the induction of a mutation. First, the chemical may have some interaction with the DNA or the enzymes involved in DNA function. While this generally results in the formation of a DNA adduct (covalent binding of the chemical with the DNA), other interactions including intercalation within the DNA strand are also possible. The inhibition of topoisomerase is another mechanism by which some chemicals cause mutations. Electrophilic chemicals that covalently bind to the DNA generally form DNA adducts containing at least a portion of the chemical. These chemical-specific DNA adducts can be identified and quantified. Such an analysis allows for a measure of the amount of primary DNA damage caused by the interaction of the chemical with the DNA. Some of these adducts can be relatively large as is the case with polycyclic aromatic hydrocarbons and heterocyclic amines. Simple alkylating agents generally form N7-methylguanine adducts. Both endogenous and exogenous reactive oxygen species form 8-oxyguanine adducts. Table 14.1 provides information about the types of DNA damage and examples of the chemicals/physical agents that cause damage and the specific mutation induced. Table 14.1 Types of DNA Damage and Examples of Chemical/Physical Agents that Induce Specific Mutations Chemical-induced DNA adducts can be repaired, misrepaired or the DNA may be processed with the adduct resulting in the incorporation of an incorrect base in the daughter DNA. DNA adducts are common. It is estimated that endogenous and exogenous chemicals can produce as many as a million molecular lesions per cell per day. Endogenous DNA damage is caused by various normal cellular processes including those that result from our reliance on oxygen for cellular metabolism and produce reactive oxidation species and misincorporation of DNA bases because of errors in replication. Exogenous DNA damage can be caused by a variety of chemical and physical agent exposures, some virtually unavoidable, including exposure to sunlight or artificial light. There are distinct differences between the types of mutations caused by endogenous and exogenous DNA damage and it is possible to provide insight into the cause of the mutational event by evaluating the DNA sequence. This pattern of mutational changes is called the DNA fingerprint or mutational spectrum. In addition, alkylating agents can induce apurinic or apyrimidinic (AP) sites because the adducts destabilize the glycosidic bond. Hydrolysis of DNA bases can cause deamination, depyrimidination, or depurination. This can occur spontaneously or from chemical exposure. The resulting AP sites can result in opening of the ribose ring and cause single DNA strand breaks. Some chemicals, particularly those containing two reactive sites, can form inter- and intrastrand cross-links in double-stranded DNA or DNA–protein cross-links. DNA cross-links can block replication and ultimately lead to cell death or to mutations in the daughter cells. For instance, carmustine (a cancer chemotherapeutic and alkylating agent) is particularly cytotoxic because it forms a cross-link between the N-1 of guanine and the N-3 of the opposing cytosine, which effectively blocks DNA synthesis and cellular replication. Cisplatin often causes intrastrand cross-links between adjacent N-7 guanines. If a break occurs in a single strand of the DNA helix, it is called a single-strand break. These are among the most common DNA lesions and generally result from attack of the DNA by endogenous free radicals and alkylating agents. Single-strand breaks also are induced by ionizing radiation and can be a secondary consequence of the repair of DNA adducts caused by a number of mutagenic chemicals. Double-strand breaks result from simultaneous breakage of the two complementary DNA strands. This can cause a physical separation of the chromosome and the loss of, in some cases, large amounts of genetic material. Such double-strand breaks will often result in cell death. Ionizing radiation and some alkylating agents induce double-strand breaks. There are a large number of human diseases that are known to involve mutations inherited from an individual’s parents. In some diseases, a single abnormal allele can cause the effect. These are referred to as dominant gene diseases. Examples of these include: Huntington’s disease, chondrodystrophy, and hepatic porphyria. Recessive mutation diseases require that the individual inherit an abnormal gene from both parents; that is, two copies of the mutated gene are required for the disease to be expressed. Diseases like albinism, cystic fibrosis, xeroderma pigmentosum, sickle cell anemia, and type 1 diabetes mellitus are recessive diseases. In hemophilia, the presence of a normal allele will block the expression of the disease. Unfortunately, the gene for hemophilia is on the X chromosome and, therefore, males who inherit a single abnormal gene from their mothers will express the disease. Females, on the other hand, have to inherit abnormal alleles from both parents. This means that hemophilia is much more common in males than females. The same is true for color-blindness, the effects of which are, of course, much less severe. Other diseases are caused by abnormal genes having low penetrance, meaning that the severity of the disease can vary among individuals who carry the mutant allele. In addition, there are diseases with complex and multiple gene involvement so that the inheritance pattern is much less clear. Anencephaly, club foot, and spina bifida are examples of these diseases. Abnormal chromosome number (aneuploidy), while generally lethal, also can result in human inherited diseases. The most well-known is probably Down syndrome in which the affected individual has three copies of chromosome 21 (trisomy). Edward’s syndrome results from trisomy of chromosome 18. Other diseases resulting from an abnormal number of chromosomes include Klinefelter’s syndrome (XXY) and Turner’s syndrome (XO; only one sex chromosome). Cri-du-chat syndrome results from a partial deletion of chromosome 5. All of these chromosome abnormalities, while having severe health impacts, are compatible with the survival of the individual. There are various estimates (up to 60% during the first trimester) as to how many spontaneous abortions occur because the fetus has an abnormal chromosome complement that is not compatible with survival. Somatic cell mutations, both inherited and newly acquired, are known to be involved in the etiology of cancer. The retinoblastoma gene (RB) is an extreme example of an inherited mutation involved in increased cancer risk. Individuals carrying an abnormal copy of the RB gene, that is, individuals who are heterozygous, need only a single mutation deleting the function of the normal allele to result in the formation of a retinoblastoma tumor. Another well-known example is the BRCA gene that dramatically increases the risk of breast cancer in women who carry a germ line mutation of this gene. Generally, the etiology of cancer involves an increase in mutation and increased cell division. In some cases, the process is initiated by a newly induced mutation. In others, some biological event causes an increased rate of cell division and this increase in the number of cells carrying a specific cancer gene mutation is thus increased in frequency. Cancer genes include both oncogenes (a mutated form of a normal gene termed a proto-oncogene), which cause abnormal cell function, and tumor suppressor genes, which when mutated cause a loss of cellular control. Some of the best characterized oncogenes include RAS, TRK, ERK, WNT, and MYC. Probably the best known tumor suppressor gene is P53. There is increasing evidence that somatic cell mutations may be involved in diseases other than cancer, including atherosclerosis, and cataracts. By the mid-1970s, there were a number of investigators who had developed tests for the detection of newly induced genetic damage. A Handbook of Mutagenicity Test Procedures was edited by B. J. Kilbey, M. Legator, W. Nichols, and C. Ramel and published in 1977 by the Elsevier Scientific Publishing Company. Chapters were written by the leaders in the newly evolving field of genetic toxicology. A wide variety of organisms, including bacteria, yeast, mouse cells, human fibroblasts, human peripheral blood lymphocytes, mice, wasps, Neurospora, onion root tips, and Drosophila were used for conducting genetic toxicology tests and most of these assays were included in the book. There were also a wide variety of endpoints, including DNA excision repair, gene mutation, mitotic recombination, micronuclei, gross chromosome aberrations, and sister chromatid exchange, described in the book. Additional chapters covered issues such as laboratory safety when working with mutagens, statistical considerations, and methods for obtaining epidemiological data for mutation risk assessment. By the 1980s, regulatory agencies were beginning to discuss the various genetic toxicology tests that might be useful for regulatory decision making. While agencies took different strategies based on regional preferences or regulatory uses and mandates, some patterns emerged. Generally, agencies were interested in selecting a combination of assays that would cover the range of adverse genetic events, including point mutations and chromosomal events. In the United States, the Environmental Protection Agency (EPA) selected a battery that included the Ames test, an in vitro mammalian assay (either the mouse lymphoma assay (MLA) using the thymidine kinase (Tk) gene or both a chromosome aberration assay and an assay using the hypoxanthine guanine ribosyltransferase (Hprt) gene) and an in vivo assay for chromosomal damage. Many agencies/organizations now have Web sites which can be readily updated and easily accessed for the latest information. The manuscript by Michael Cimino (2006), listed in the suggested reading section, provides a useful summary. The Organization for Economic Cooperation and Development (OECD) has published guidelines for the commonly used genetic toxicology assays. An expert workgroup was convened within the last couple of years and this group is currently revising the test guidelines. As indicated earlier, the standard battery usually includes a bacterial assay, an in vitro mammalian cell assay capable of detecting chromosomal damage and an in vivo cytogenetic assay. All of the in vitro assays must be conducted both with and without exogenous metabolic activation because the cells used have limited capability to metabolize promutagens to their active metabolites. Generally, this activation is accomplished using a cofactor-supplemented S9 fraction prepared from the livers of rodents (generally rats) that have been treated with a chemical such as Aroclor 1254 or a combination of β-naphthoflavone and phenobarbital. The S9 used for the in vitro genetic toxicology assays is a mixture of microsomes and cytosol. It contains a variety of both Phase I and Phase II enzymes and includes cytochrome P450 enzymes, acetyltransferases, carboxyesterases, and other enzymes. Some investigators have developed methods whereby primary hepatocytes are cocultured with the mammalian cells used in genotoxicity assays, and it is possible to make S9 from a variety of species including humans. As a general rule, these latter activation methods are more common in research applications and are rarely used in routine in vitro genetic toxicology evaluations. The most widely used genetic toxicology assay is the Ames test, which uses a set of specially designed strains of Salmonella typhimurium (Figure 14.5). The Ames test is commonly used as an initial screen to determine the mutagenic potential of chemicals. The assay detects point mutations using a set of tester strains containing preexisting mutations that require the presence of histidine in the medium in order to grow. Mutations that restore a functional sequence to the gene enable the bacteria to synthesize histidine. These mutations that change a bacterium back to function are called reversion mutations and the mutated bacteria, revertants. Figure 14.5 The Ames test is conducted by treating the various tester strains of Salmonella with the test chemical, plating in petri dishes and counting the number of revertants that can grow and form colonies. Each of the tester strains has its own properties, having different types of mutations that must be reverted to histidine independence in order to grow into colonies that can be enumerated (Table 14.2). By using a set of these strains it is possible to detect and distinguish mutagens acting by various mechanisms. Some of the strains are reverted by base pair substitutions. Others contain frame shift mutations and therefore require frame shift mutations to restore function. Because bacteria have cell walls that are not as permeable as the cell membranes of mammalian cells, all of the strains contain mutations that have damaged the cell wall in a way that allows test chemicals to enter the bacteria. In addition, some of the strains contain defects in DNA repair enzymes so that any initial DNA damage will not be repaired, but rather will result in revertants that can be grown into colonies which can be scored. Table 14.2 Commonly Used Ames Test Strains, the Types of Mutation Detected and Example Mutagens for Each Strain To conduct the assay, the bacteria are plated with a trace amount of histidine in petri dishes containing agar. There are three basic versions of the assay: the spot test, the plate incorporation test, and a version in which the chemical and bacteria are preincubated and then plated to enumerate the revertants. There are some strains that are engineered to express some metabolic capability, but in general the bacteria have little to no metabolic ability and it is necessary to use an exogenous metabolic system generally made from rat liver. Typically, the assay is conducted both with and without S9. This provides some information as to whether the chemical is itself capable of inducing mutation or if metabolism to active metabolites is a prerequisite for the chemical to induce mutations. In addition to the Ames test, the Escherichia coli assay is sometimes used and is based on mutations in the tryptophane biosynthetic pathway. Both of these bacterial assays detect point mutations involving single or a very small number of base pairs and include substitutions (transitions and transversions), and the addition or deletion of one or a few base pairs. Therefore, a complete assessment of a chemical requires that additional tests be performed using mammalian cells and endpoints that detect both gene mutations and chromosomal events. The MLA using the thymidine kinase gene (Tk) is the most widely used in vitro mutation assay (Figure 14.6). The next most widely used in vitro gene mutation assays utilize the Hprt locus. Because the Hprt gene is located on the X chromosome, it is possible to conduct the Hprt assay in most cell types. The Tk locus, on the other hand, is located on an autosome (chromosome 11 for mice, chromosome 17 for humans) and so it is necessary to generate a Tk heterozygous line in order to detect mutation from Tk+/− to Tk−/−. Figure 14.6 The MLA is conducted by treating cells with the test chemical, removing the chemical, resuspending the cells, and allowing the newly induced mutations to express. Following a 2-day expression period, the cells are cloned at a high cell density with the selective agent so that the mutants can grow and at a low cell density to determine plating efficiency. Following a 10- to 12-day incubation, the colonies are counted, mutant colony size determined, and mutant frequency calculated. In the early 1970s, Donald Clive and coworkers isolated a Tk+/− subline for the specific purpose of developing a mammalian cell mutation assay. This was accomplished using bromodeoxuridine to select clones that were deficient in the Tk enzyme. The growth of cells that have Tk enzyme is arrested, while cells that have no Tk enzyme are capable of growing in the presence of the selective drug and can form into colonies. Because the frequency of Tk-deficient cells is very low (on the order of 1 × 10−9) in a population of Tk+/+ cells, a very large number of cells were required to obtain a small number of Tk−/− clones. These Tk-deficient clones were expanded and allowed to grow into a population of cells. To obtain the Tk+/− clones required for the mutation assay, a selective medium containing methotrexate was used to yield clones that had a reversion of one Tk− allele to Tk competency. Ultimately, a subline with the designation L5178Y Tk+/− 3.7.2C was developed and is now used routinely. While the assay was originally developed using bromodeoxyuridine as the selective agent, a better selective agent was soon identified. Trifluorothymidine (TFT) was shown to provide for more rapid arrest of Tk-enzyme-containing cells and therefore to provide for better growth and more accurate enumeration of Tk mutant cells. Now, TFT is the only approved selective agent for use with the MLA. The assay is conducted using a range of test chemical concentrations that cover a prescribed cytotoxicity range. The MLA was developed using a unique measure of cytotoxicity, the relative total growth (RTG), which takes into account the toxicity during the cell treatment and culture and also during the cloning phase of the assay. The recommended maximum cytotoxicity is between 20 and 10% RTG, which does not represent a “killing” of 80–90% of the cells. In fact, it is similar to the maximum cytotoxicity now recommended for the in vitro cytogenetic assays (see OCED TGs 473 and 487) for most chemicals. Because newly mutated cells require time to become deficient in the Tk enzyme, a period of time called the phenotypic lag or expression time is required before the mutant frequency can be enumerated. For the Tk gene, it has been determined that 2 days are sufficient and optimal. It is important to note that longer expression times are not acceptable because the mutant frequency declines. Following the 2-day expression period, cells are plated at high cell density in medium containing TFT that will allow only Tk mutants to grow and also at a very low cell density to determine the plating efficiency of the cells. The mutant frequency is calculated based on the number of mutants that grow out of the population of cells plated in the TFT, corrected for the plating efficiency (i.e., the percentage of cells that can grow in the cloning plates). The plates are incubated for a sufficient time (about 10–12 days) to allow the colonies to attain sufficient size for counting. Mutant frequency is usually expressed as the number of mutants per million cells. The normal background (nontreated culture) mutant frequency is between 35 and 170 Tk mutants per million cells.
MUTAGENESIS AND GENETIC TOXICOLOGY
14.1 FUNDAMENTALS OF CHEMICALLY INDUCED GENETIC DAMAGE
Type of DNA Damage
Causative Agent
Major Mutation
DNA hydrolysis products
Deaminated bases
Spontaneous deamination of 5-methylcytosine
G:C→A:T transition
Apurinatic lesions
Alkylating agents
G:C→T:A transversion
Apyrimidinic lesions
Alkylating agents
A:T→ T:A transversion
DNA monoadducts
8-oxoguanine
Reactive oxygen species
G:C→T:A transversion
Alkyl adducts
Alkylating agents (e.g., ENU), spontaneous methylation
Base pair substitution
Bulky adducts
PhiP, Benzo[a]pyrene, N-2-actylaminofluorene
Base pair substitution, frame shift
Cross-links
Thymine dimmer
UV light, mitomycin C
Tandem mutation, base pair substitution
Interstrand crosslinks
Bifunctional alkylating agents (e.g., carmustine)
Frame shift, deletion, chromosome mutation
Intrastrand crosslinks
DNA and protein crosslinks
Cisplatin, aldehydes
Basepair substitution
Chromosome mutation
Single-strand breaks
Free radicals, ionizing radiation, UV, alkylating agents
Deletion, insertion, base pair substitution
Double-strand breaks
Ionizing radiation
LOH, deletion, translocation
Nondisjunction
Colcemid
Aneuploidy
14.2 GENETIC DAMANGE AND ITS IMPACT ON HUMAN DISEASE
14.3 GENETIC TOXICOLOGY TESTS
Assays in the Standard Battery
Bacterial Reverse Mutation Assays (OECD TG 471, FDA Redbook)
Strain
Mutation Detected
Target Sequence
Mutagen
TA97
Frame shift
CCCCCC
4-NQO
TA98
Frame shift
CGCGCGCG
2-AF
TA100
Base pair substitution
GGG
BaP, MMS
TA102
Base pair substitution
TAA
H2O2, MMC
TA104
Base pair substitution
TAA
formaldehyde
TA1535
Base pair substitution
GGG
2-AA, NaN3
TA1537
Frame shift
CCCCC
2-AA, AAC
TA1538
Frame shift
CGCGCGCG
2-NF
In Vitro Mammalian Cell Gene Mutation Assays (OECD TG 476, FDA CFSAN Redbook)
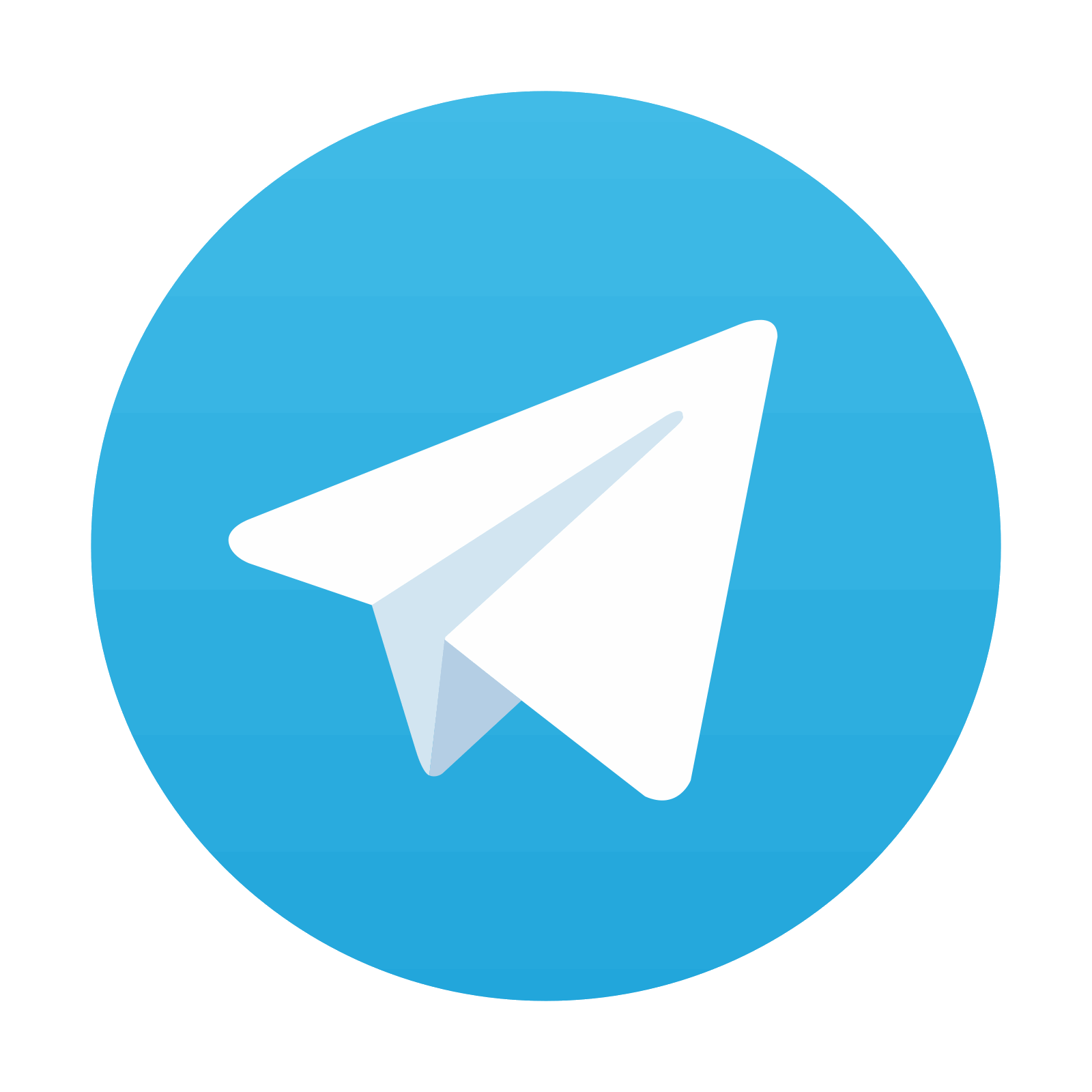
Stay updated, free articles. Join our Telegram channel
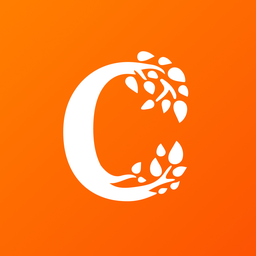
Full access? Get Clinical Tree
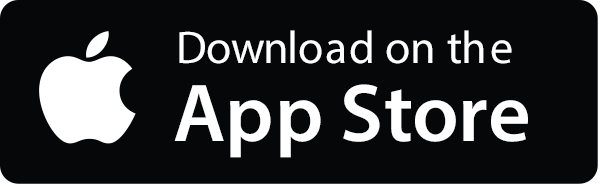
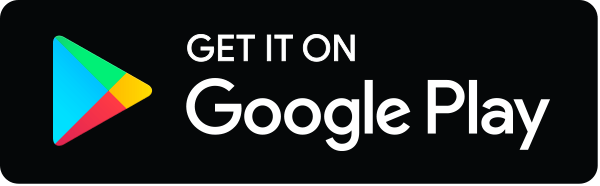