Chapter 5
Multimodal Morphochemical Tissue Imaging
Modern optics and spectroscopy are offering promising non-invasive solutions to potentially improve diagnostic capability on tissues, as demonstrated by the extensive use of non-linear laser scanning microscopy for tissue imaging in the past decade. The recent development and integration of multiple non-linear microscopy techniques in a single instrument has provided new opportunities for integrating morphological and functional information and for correlating the observed molecular and cellular changes with disease behaviour. In particular, multimodal non-linear imaging is able to perform a morphochemical quantitative analysis in tumour cells and tissue specimens, providing a high-resolution label-free alternative to both histological and immunohistochemical examination of tissues. Although up to now limited to optical research labs, multimodal non-linear imaging is becoming increasingly popular among medical doctors and has the potential to find a stable place in a clinical setting in the next future.
5.1 Introduction
The “gold standard” for tissue diagnostics is represented by the histological exam, which is commonly performed by means of white-light optical microscopy on cryosectioned, processed, and labeled slices of tissue. The largely used hematoxylin and eosin (H&E) staining allows tissue classification by means of a morphological analysis, while chemical analysis generally requires the use of specific labels and fluorescence microscopy in an immunohistochemical approach. In both cases, tissue samples undergo a long procedure, including dehydration, fixation, inclusion, labeling, and observation. In this view, an alternative or a complementary technique able to provide similar information with respect to that provided by common histology in a fast, reliable, and label-free way would be desirable.
Nonlinear optical (NLO) microscopy is offering promising solutions for deep tissue imaging at a subcellular level without any exogenous probe and can provide both morphological and functional information on the sample under investigation. NLO microscopy offers several advantages with respect to other single-photon laser scanning imaging techniques. First, the nonlinear dependence of the signal on the excitation light intensity allows the selective excitation of only molecules located in an extremely confined volume around the focal point. The direct consequence is an intrinsically high spatial resolution in comparison with other conventional microscopy techniques that employ similar excitation wavelengths. Second, the axial confinement of the excitation volume intrinsically provides optical sectioning capability, as the interaction with molecules located “out of focus” is prevented. This feature allows reducing photodamage and phototoxic effects with respect to confocal microscopy. In fact, a confocal pinhole that rejects out-of-focus light is not required in NLO microscopy, allowing for a 3D scanning of the specimen using a reduced exposure compared to confocal microscopy. Third, NLO microscopy employs near-infrared (NIR) laser wavelengths for exciting electronic transitions with absorption bands located in the visible region of the spectrum. This feature offers deep label-free imaging in biological tissues [1, 2]. In fact, every biological tissue intrinsically contains a certain amount of fluorescent molecules with excitation bands located in the visible range. This allows the imaging of biological tissue without adding any probe. Nevertheless, light penetration into optically turbid samples is strongly limited by scattering and absorption when using wavelengths in the visible range so that imaging inside biological tissue is limited to the outermost layers, whereas deep imaging is a fundamental issue for tissue characterization. In NLO microscopy, the use of NIR wavelengths offers the advantage of reduced scattering compared to linear microscopy and hence a deeper penetration inside optically turbid samples such as biological tissues. Further, scattered photons do not contribute to the excitation because they are unable to reach the excitation volume. This results in a spatial resolution that remains almost unchanged when imaging deep into an optically dense specimen [3]. For these reasons, NLO microscopy allows label-free optical sectioning with sub-diffraction-limited resolution in deep tissue.
NLO microscopy includes several laser scanning imaging techniques. The common feature to all the techniques is the nonlinearity of the interaction between light and the biological specimen, which is mediated by two or more photons. Although they can be divided in the two main groups of coherent and incoherent techniques, both of them are able to provide not only morphological but also chemical information on the tissue under investigation. The combination of NLO microscopy techniques providing morphological information with those providing functional information is crucial for tissue classification and pathological assessment. In fact, an important feature in understanding the development of a tissue disease is the relationship between morphological features such as architecture, shape, symmetry, and functional features. We believe that Nature chooses the best shape and organization to accomplish a certain function. The relationship between morphology and function has to be strict, even if complex. The proper combination of NLO microscopy techniques in a morpho-chemical multimodal imaging approach has the potential to image and classify tissues in a fast and reliable way, as well as to provide new insights into the understanding of tissue diseases in a label-free modality. Hence, such a multimodal approach has the potential of being used in vivo.
5.2 Morphological Techniques
5.2.1 Two-Photon Excited Fluorescence (TPEF) Microscopy
Among the incoherent techniques that are able to provide morphological information, two-photon excited fluorescence (TPEF) microscopy is the most popular and widespread NLO microscopy technique. TPEF is based on the possibility to excite an electronic molecular transition, instead of through absorption of a single photon with frequency in resonance with the transition, by simultaneous absorption of two photons, each of them having one-half the transition energy. Such a process was first theorized in 1931 by Goppert-Mayer [4]. Thirty years later, Kaiser and Garrett [5] first observed a two-photon excited transition in a crystal. The implementation of the technique in a laser scanning microscope occurred only in 1990 with the work of Denk et al. [6]. The strong potential of this microscopic technique is demonstrated by the growing number of studies in both biological and biomedical fields employing TPEF microscopy [1, 7, 8]. In the last 20 years, it has been successfully used in a broad range of applications in life sciences [9–13]. TPEF microscopy is particularly powerful for tissue imaging. In fact, it can provide morphological information of the tissue under investigation with subcellular resolution at both the epithelial and connective tissue level by taking advantage of the endogenous fluorescence of nicotinamide adenine dinucleotide hydrogenase (NADH), flavin adenine dinucleotide (FAD), and elastic fibers [14, 15]. Several studies have also demonstrated the potential of TPEF microscopy for in vivo tissue imaging. Up to now, the most popular in vivo applications of TPEF microscopy have been in imaging skin of living subjects [16–19] and imaging brain of living small animals [2, 20]. In particular, dermatological imaging using TPEF microscopy is now possible in a clinical setting [21–23], even though much work has to be done before its recognition as a standard diagnostic technique. The next challenge is represented by the implementation of TPEF microscopy in endoscopy. Although TPEF and other NLO endo-microscopes have been already developed within research labs [24–27], several improvements have to be made in terms of imaging speed, probe size, and axial scanning capability in order to see a TPEF endoscopic microscope to be routinely used for clinical diagnostic imaging.
5.2.2 Second-Harmonic Generation (SHG) Microscopy
Another powerful NLO imaging technique that is able to provide morphological information of some peculiar tissue constituents is represented by second-harmonic generation (SHG) microscopy [28, 29]. SHG microscopy is a coherent microscopic modality capable of providing background-free images of anisotropic biological molecules having large hyperpolarizability [30, 31], such as collagen [32–34], muscle [35–40], or microtubules [41]. SHG microscopy is based on a nonlinear second-order scattering process that occurs in specimens satisfying particular requirements. At the molecular level, the requirements are satisfied by molecules having a large hyperpolarizability. Although several biological molecules are rich in molecular systems that can potentially generate SHG, an additional requirement related to the structural organization of single molecular emitters at the focal volume scale needs to be satisfied in order to have a detectable SHG signal. It consists in having structural anisotropy at the focal volume scale. Both conditions are satisfied in anisotropic biological molecules such as collagen, muscle, or microtubules, making SHG microscopy an ideal tool for imaging and probing the organization of these biological systems. During the last years, SHG microscopy has been successfully applied to the imaging of noncentrosymmetric molecules inside cells [14, 42, 43], cellular membranes [44–48], brain [41], and biological tissues [15, 49, 50]. The capabilities of SHG microscopy in providing background-free, high-resolution imaging of collagen-rich tissues have already been largely demonstrated [51] in various tissues such as cornea [52, 53], tendon [54, 55], and arteries [56]. In fact, a single collagen molecule has a high hyperpolarizability, and the supramolecular organization in fibrous structures [57] provides an extremely strong SHG signal [32]. In this way, collagen can be selectively imaged with high resolution in connective tissues by means of SHG microscopy [15, 58]. SHG microscopy can be used for the investigation of both structural organization and fibrillar orientation of collagen in human dermis [59–63], keloid [64–66], fibrosis [67–69], thermally treated samples [70–74], and also in tumor microenvironments [75–81]. Because of its sensitivity to the organization of molecular emitters, SHG microscopy can be used to detect altered physiological conditions of various kinds of tissues, including muscles [82], bones [83, 84], and cartilages [85, 86]. In fact, an altered organization of molecular emitters can be an indicator of a diseased condition of the tissue. Further, the investigation of collagen morphology in the connective tissues and in the tumor environment is relevant from the diagnostic point of view. In fact, collagen organization in the region surrounding tumor lesions can be related to the tumor invasiveness and plays a crucial role in the development from an in situ to an invasive stage.
SHG and TPEF microscopy can be implemented in the same microscope, using the same laser source, to provide a powerful tool for imaging both cells and connective tissues. While TPEF microscopy provides high-resolution imaging of intrinsic molecular signals in living cells, SHG enables the direct imaging of collagen. The combination of SHG and TPEF is particularly powerful for imaging connective tissue. In fact, the main components (collagen and elastin) can be imaged by SHG and TPEF microscopy, respectively. Multimodal TPEF–SHG microscopy has been successfully used for characterizing physiological and pathological conditions of connective tissues. They have been mainly applied to dermal imaging for studying cutaneous photo-aging [22, 87–89] and skin tumors [62, 70, 90]. Changes in the intensity of intrinsic fluorescence and morphologic changes in collagen structure imaged by SHG can be primary indicators of diseased states. The combination of these two techniques is a powerful solution for imaging tissue morphology without labeling, but they are not able to provide functional information unless used in combination with additional microscopic modalities.
5.3 Functional Techniques
5.3.1 Fluorescence Lifetime Imaging Microscopy (FLIM)
TPEF microscopy is generally based on the measurement of a steady-state fluorescence signal. Using this approach, tissue characterization is performed by simply detecting an optical contrast in terms of fluorescence intensity between the lesion and the surrounding healthy tissue. Such contrast can be obtained by detecting different fluorescence levels between healthy and diseased tissue, which is generally due to the relatively different native fluorescence of the two tissue types. The contrast level achievable with this approach is in general not very high, leading to difficulty in discriminating diseased tissue, especially at the premalignant or early stage. In addition, several causes can contribute to the fluorescence level detected, including a different concentration of endogenous fluorophores, a different tissue morphology that affects the propagation of light, and a different microenvironment potentially affecting the quantum yield of the excited fluorophores. Further, as discussed in the previous section, this approach can provide only morphological information.
If using TPEF microscopy, functional information can be obtained by the spectral and temporal analysis of the fluorescence emission. In particular, a major improvement in fluorescence investigation is represented by the analysis of the fluorescence dynamics. This approach consists in creating image contrast using the fluorescence lifetime instead of the fluorescence intensity as in steady-state measurements. Lifetime measurements offer several advantages in comparison to steady-state measurements. First, fluorescence lifetime is independent of light losses within the tissue. Second, fluorescence lifetime of a molecule is very sensitive to its microenvironment and to energy exchanges, so that a different fluorescence lifetime in a tissue with respect to a control condition can be taken as an indicator of an altered physiological condition. Finally, fluorescence lifetime is almost independent of the excitation intensity, so that lifetime measurements can be used to overcome the limitations caused by intensity fluctuations that strongly affect steady-state fluorescence measurements. Endogenous fluorescent molecules have typical fluorescence lifetimes in the range of hundreds of picoseconds to a few nanoseconds and they can be measured by means of fluorescence lifetime spectroscopy techniques. The implementation of this technique in a microscopic approach that is able to map fluorescence lifetime is commonly known as fluorescence lifetime imaging microscopy (FLIM). FLIM can be implemented in a laser scanning microscope equipped with a pulsed illumination source and a fast-response detector. It has already been demonstrated to be a powerful technique in providing functional information about tissue conditions [19, 23, 91–95]. In particular, FLIM has been successfully employed to characterize various tissues and to probe cellular differentiation in the epithelium, as demonstrated by studies performed on cell cultures [96], fresh biopsies [97], and also in vivo [21, 98]. An additional powerful application of FLIM consists in the possibility to optically detect variations in the fluorescence lifetime of endogenous fluorescent molecules involved in cellular metabolic pathway, such as NADH and FAD [99]. In particular, FLIM is able to detect whether the two nucleotides NADH and FAD are in their free or protein-bound state. Changes in the fluorescence lifetimes of these two nucleotides can reflect changes in their binding sites, which can potentially be related to altered metabolic conditions characteristic of dysplasia or neoplasia. Hence, this approach has the potential to detect a tumor at a very early stage.
5.3.2 Multispectral TPEF Imaging (MTPEF)
Another approach that is useful for detecting functional information when using TPEF microscopy, and is complementary to the analysis of fluorescence decay, is represented by the spectral analysis of the fluorescence emission in a multispectral approach. Multispectral two-photon excited fluorescence (MTPEF) microscopy can be implemented in a two-photon laser scanning microscope by detecting the fluorescence signal on multiple spectral channels. This can be obtained by placing in the detection path a spectral separating element (i.e., a prism or diffraction grating) and a multichannel detector such as a photomultiplier strip or a charge-coupled device (CCD) camera instead of a single-channel detector [100]. The MTPEF microscopy approach allows the discrimination of various fluorescent molecules inside the tissue based on their fluorescent spectra, upon spectral unmixing of the detected signal. MTPEF imaging has been applied to the imaging and characterization of cells [101, 102] and various kinds of tissues, especially skin [103–108] and the gastric tissue [109]. The selective spectral excitation and detection of NADH and FAD fluorescence is of particular interest for diagnostic purposes. This can be accomplished by means of MTPEF microscopy using two particular excitation wavelengths for selectively exciting NADH and FAD endogenous fluorescence, and two spectral channels for detecting the corresponding fluorescence. This approach can provide functional information about the cellular metabolic condition of the tissue under investigation, which can in turn be related to diseased conditions, such as cancerous or precancerous.
5.3.3 Raman-Based Microscopy (Raman–CARS–SRS)
Additional and more detailed characterization in terms of functionality can be obtained by using Raman-based techniques aimed at imaging tissue and resolving the vibrational structure of molecules simultaneously. Raman-based microscopy techniques provide a means for label-free imaging of biological specimens and tissues in a morpho-chemical approach. In fact, every biological specimen is characterized by a vibrational spectrum representing a chemical-specific fingerprint of its molecular components [110]. Spontaneous Raman scattering microscopy is the most widely used vibrational microscopy technique. It provides label-free chemical information as vibrational spectroscopy with the advantage of intrinsic high spatial resolution due to the shorter wavelengths used with respect to the transition under investigation. The sensitivity of spontaneous Raman microscopy to the vibrations of particular molecules allows the investigation of the molecular structure of analytes, providing a label-free morpho-chemical characterization of a biological specimen. As this technique is based on the inelastic scattering of light, the detection can be performed in the backscattering geometry, making in vivo clinical applications possible. Nevertheless, spontaneous Raman microscopy has strict limitations in terms of both sensitivity and imaging speed. In fact, because of its low cross section, this technique does not have enough sensitivity to be used for imaging at high speeds. In addition, as a linear technique, spontaneous Raman microscopy does not provide the above-described advantages of NLO microscopy.
A significant step forward has been made with the implementation of coherent anti-Stokes Raman scattering (CARS) microscopy [111]. CARS microscopy represents a nonlinear variant of spontaneous Raman scattering. It uses two synchronized, pulsed laser sources (pump and Stokes) properly tuned in order that their frequency difference coherently drives the vibrational transition to be investigated. The illumination with a third pulsed source (probe), which is in general taken from the pump source, interrogates the sample, generating an anti-Stokes signal which is blue-shifted with respect to both the pump and Stokes beams. This technique allows the investigation of the distribution of various molecular vibrations by properly tuning the pump and Stokes frequency to match the molecular transition to be investigated. CARS also offers the possibility of chemical characterization of tissues by providing vibrational spectroscopy of chemical bonds at the level of optical resolution [112–114], but with increased sensitivity compared to spontaneous Raman spectroscopy, thus enabling imaging at higher speeds. On the other hand, CARS microscopy is generally used to perform imaging of a specific vibrational transition and does not provide the exhaustive information of a full Raman spectrum, unless the frequency difference of the pump and Stokes beam is swept over a broad range. Moreover, CARS suffers from a strong nonresonant signal background due to the instantaneous third-order nonlinear response of the medium, which causes complication in image interpretation and limits the detection sensitivity, especially when imaging in a biological tissue. In most studies, single-band CARS has been used to image the distribution of the symmetric and asymmetric C–H stretching vibration [115–117], which is the main component in lipids. CARS microscopy offers great potential for both ex vivo [118–120] and in vivo clinical diagnostics [114, 121] by providing a specific technique for imaging lipids. In addition, other relevant biological molecules yield good CARS image contrast, for example, DNA or proteins [122, 123].
Recently, a new Raman-based microscopic technology has been developed to overcome the limitations of both the low sensitivity of spontaneous Raman and the nonresonant background of CARS. Stimulated Raman scattering (SRS) microscopy provides label-free chemical contrast inside biological tissues with high spatial resolution [124–129]. SRS is based on two pulsed laser sources which provide the pump and Stokes beams. In SRS microscopy, the Stokes beam induces the depletion of the excited virtual level by means of stimulated emission. This optical process induces an increase in the intensity of the output Stokes beam (stimulated Raman gain, SRG) as well as a decrease in the intensity of the output pump beam (stimulated Raman loss – SRL). SRS can be detected by measuring SRG or SRL. For picosecond-pulsed lasers, the detection sensitivity of both SRG and SRL is on the order of 10−4. A dramatic increase of detection sensitivity (up to 10−7) can be obtained with a high-frequency phase-sensitive detection scheme. This detection technique consists in modulating the Stokes beam intensity at a frequency on the order of megahertz and detecting the pump beam intensity via a lock-in amplifier. Lock-in detection provides background-free SRL by removing low-frequency laser noise and intensity fluctuation and pushes the detection sensitivity of SRS to a value of up to 10−7. Preliminary results on living cells and fresh tissues have demonstrated the capability of SRS in monitoring cellular uptake of fatty acids, as well as in imaging lipids in tissues. Its use has been also tested on fresh skin specimens, revealing not only the possibility to image lipids in skin but also to monitor the transdermal delivery of exogenous agents such as dimethyl sulfoxide (DMSO) or retinoic acid [125].
Despite the power of NLO microscopy techniques for tissue imaging, their clinical application has so far been limited to TPEF, SHG, and FLIM techniques. The implementation of combined morphological and functional techniques in a multimodal approach has so far been confined to high-tech research laboratories because of the complexity and cost of the laser systems typically used for generating the pump and Stokes beams. Nevertheless, we believe that these complex microscopic setups will be available in the mainstream biomedical, biochemical, and clinical research laboratories in the near future.
In this chapter, after having provided a detailed description of both morphological and functional nonlinear imaging techniques (Section 5.1), we describe some applications of these techniques to tissue imaging. In particular, we first review applications involving morphological imaging using both TPEF and SHG microscopy (Section 5.4). Then we present some relevant imaging applications more devoted to the morpho-functional imaging, employing MTPEF and polarization scanning SHG microscopy (Section 5.5). Finally, in the last part of Section 5.5, we describe a morpho-chemical characterization of tissue using a multimodal approach combining TPEF, SHG, and CARS microscopy. At the end, we summarize conclusion and potential future application of the multimodal, nonlinear imaging approach.
5.4 Morphological Imaging
TPEF microscopy is particularly powerful for tissue imaging. In fact, TPEF microscopy can provide morphological information of the tissue under investigation with subcellular resolution at both epithelial and connective tissue level by taking advantage of the endogenous fluorescence of NADH, FAD, and elastic fibers, respectively. SHG microscopy is extremely powerful for imaging connective tissue. In fact, collagen is the most important component of connective tissue, and it provides a strong SHG signal due to its high molecular hyperpolarizability and its fibrillar organization. SHG is a fantastic tool for imaging collagen and probing its hierarchical organization from molecular scale up to tissue architectural level. In this section, we present three different applications of tissue imaging employing TPEF and SHG microscopy aimed at providing morphological characterization. In particular, we first describe the possibility to quantitatively analyze cellular morphology in healthy and pathological bladder tissue by taking advantage of TPEF emitted by NADH. Then we present a morphological scoring of collagen organization using image pattern analysis of SHG images. Finally, we include a multimodal TPEF–SHG morphological imaging approach aimed at characterizing dermal tissue in physiological and pathological conditions.
5.4.1 Cell Imaging by TPEF Microscopy
Cellular morphology can be highlighted with high resolution by taking advantage of the endogenous TPEF signal provided by NADH. Imaging can be performed in both living tissue and fresh biopsies as NADH can be found inside freshly excised tissues up to 4–5 hours from excision [15, 130]. In this example, TPEF imaging was performed on fresh bladder biopsy samples within 2–3 hours of surgery using an excitation wavelength of 740 nm, which is adequate for exciting NADH fluorescence by two-photon absorption. Thick tissue samples can be optically sectioned with subcellular resolution using TPEF microscopy, thereby enabling high-resolution imaging of epithelial cells. Samples containing both healthy mucosa (HM) and carcinoma in situ (CIS) were examined in this study [131] with the aim of providing morphological characterization of both healthy and pathological tissue. The TPEF images in Figure 5.1a,c demonstrate a good correlation with the corresponding histological images (Figure 5.1b,d), taken after histological examination of the same samples. NADH is located inside cytoplasm, so NADH fluorescence allows visualizing both healthy and cancer cells with a strongly fluorescent cytoplasm and a dark nucleus, as shown in Figure 5.1a,c. It is clearly visible that cancer cells have large nuclei and small cytoplasm. On the other hand, healthy cells show larger cytoplasm and smaller nuclei. This morphological signature of tumor cells has already been measured by TPEF microscopy in other tissues, such as skin [90], and it could be taken as a typical signature of malignancy. This feature is crucial for performing an analysis of the cell morphology. In particular, by means of a proper image segmentation algorithm based on the fluorescence level, it is possible to quantitatively assess the size of both the cell and the nucleus. A quantitative scoring of such morphological feature can be obtained by considering the cellular-to-nuclear size ratio, which represents a dimensionless index independent of cellular size. In segmented TPEF images, both the cellular and nuclear borders are highlighted, allowing the determination of the cellular and nuclear size and hence the calculation of their ratio. The obtained results for this example involving bladder tissue are plotted in a bar histogram in Figure 5.1e. CIS cells have a smaller ratio compared to HM cells, in agreement with this typical signature of malignancy. Such kind of analysis can be easily extended to other tissues, as NADH is present in every kind of epithelium. The extension of this approach to other inflammatory and dysplastic tissue conditions could open the possibility to use this method as a score for diagnosing tumors at a very early stage.

Figure 5.1 TPEF (green-coded) images taken from human ex vivo fresh biopsies of bladder and the corresponding histological images taken after H&E staining of the same samples. An optical section of healthy mucosa (a,b) acquired at 25 μm depth from tissue surface. An optical section of CIS (c,d) acquired at the same depth as above. Scale bars: 10 μm. (e) Histogram distribution of the cellular-to-nuclear area ratio for CIS (in red) and for HM (in black), calculated by summing over images acquired in a 20–30 μm, on 5 samples of CIS and 5 samples of HM and over 10 cells per sample.
5.4.2 Collagen Organization Probed by Pattern Analysis of SHG Images
Scoring the organization of collagen fibers is crucial for providing an exhaustive description of tissue architecture. Several methods have already been developed aimed at characterizing collagen at the tissue architectural level. These methods can be grouped in the two major groups, namely, geometrical methods and statistical methods. In this section, we describe one example applied to the characterization of various collagen networks.
Geometrical methods are based on automated pattern recognition of relevant geometrical shapes within the observed collagen network. A first example aimed at evaluating the geometrical properties of collagen fibers in normal skin dermis and keloid was reported in [66]. In this work, the authors used both the waviness and orientation of collagen fibers as geometrical parameters to discriminate keloid from normal human dermis. Both parameters were then implemented into an algorithm with the final aim of providing a reliable scoring method. The algorithm developed consisted in a series of calculations on the acquired SHG images, including contrast enhancement, image filtering, and segmentation. The overall procedure allowed the detection of collagen fiber edges from which the geometrical parameters could be extracted. Another geometrical approach exploited consisted in determining the volume occupied by collagen fibers [67, 132]. This method offers the advantage of being applicable to 3D reconstructions of collagen networks, and was successfully employed for scoring connective tissue fibrosis in an animal model. The algorithm developed consisted in an image processing routine, including devignetting and denoising procedures, that allowed the definition of three different scores. By analyzing several regions in a sample with fibrotic kidney tissue, the authors found that the three scores allowed the discrimination of different tissue properties as well as the identification of both treated and control samples.
Statistical methods are generally based on fast Fourier transform (FFT) analysis or on gray-level co-occurrence matrix (GLCM). FFT analysis allows the retrieval of information on the spatial frequencies contained in an image, which are in turn related to the original pattern contained in the image. Considering the fibrillar structure of collagen-rich tissues, FFT-based methods are particularly suitable for detecting structural anisotropy of fiber distribution. In fact, the mean orientation of collagen fiber distribution in the original image is reflected in the shape of the FFT profile. Randomly oriented fibers result in an FFT profile with circular behavior, whereas, as the fibers become more oriented, the profile becomes elliptic with increasing eccentricity. The aspect ratio of the FFT profile can be taken as a score of anisotropy for the original image. Such a method has been used for characterizing various collagen-rich tissues such as cornea [71] and skin [64, 133], and has the advantage of providing an intensity-independent scoring method offering a scalable modality to provide information at various scale levels. GLCM is another simple and well-established approach for analyzing the SHG images of collagen. The method provides multiple scores related to the spatial relationships between pixel intensities in the original image. The scores are generally grouped in contrast and orderliness, and are based on statistical methods. Among the statistical GLCM methods, correlation provides a powerful score for characterizing SHG images of collagen, as it provides information on the repetition of a characteristic pattern in the image. GLCM correlation measures the intensity relationship between pixel couples in the image. If two pixels give a high value of correlation, this means that their intensities are consistent with a well-defined relationship. The calculation is performed by considering all the possible pixel couples in the image. By analyzing correlation versus offset (distance between the analyzed pixel couple), it is possible to retrieve information on the periodicity of a certain pattern as well as on a sudden change of a regular structure. GLCM correlation was already successfully used to determine, in good agreement with physiological values, the mean size of collagen fiber bundles in healthy dermis (HD) and keloid [64]. An example of a GLCM correlation analysis is shown in Figure 5.2. Here, the GLCM correlation analysis allowed characterizing, in terms of the size of the collagen fiber bundles, three different collagen networks, namely artificial collagen, healthy skin, and keloid. The method was recently improved through the calculation of GLCM correlation upon rotation of the original image in an orientation-dependent gray-level co-occurrence matrix (OD-GLCM) approach [134], demonstrating the capability of the method in assessing collagen fiber bundle size during pathological development.

Figure 5.2 SHG images of collagen fiber bundles within a sample of (a) artificial collagen, (b) healthy dermis, and (c) keloid. (d) The corresponding results for the GLCM correlation plotted versus neighbor distance, expressed in micrometers for artificial collagen (blue triangles), healthy dermis (black squares), and keloid (red circles).
5.4.3 Multimodal TPEF–SHG Microscopy of Skin Dermis
NLO microscopy, in particular combined TPEF–SHG microscopy, is a fantastic tool for performing morphological analysis of connective tissue and characterizing physiological and pathological states. In fact, the architectural features of connective tissues are mainly due to the abundance and organization of elastic and collagen fibers. The combination of the two techniques is ideal for connective tissue imaging. In fact, while SHG microscopy allows direct imaging of collagen, elastic fibers have a strong TPEF cross section and emit fluorescence under two-photon excitation. The combined TPEF–SHG technique has the potential to be used also in vivo for clinical diagnostic imaging, as it offers penetration depth capability in thick tissue up to 200 μm. When employing combined TPEF–SHG microscopy, a simple characterization method is represented by the second-harmonic to autofluorescence aging index of dermis (SAAID). This scoring method is simply based on calculating the normalized ratio between SHG and TPEF signals, which is in turn related to the relative abundance of collagen versus elastic fibers. It was first introduced by Lin et al. [87] for measuring skin aging and has been applied to the evaluation of intrinsic and extrinsic aging of both ex vivo [87] and in vivo [22] skin dermis. In fact, it is known from histology that skin aging is characterized by a reduced collagen abundance and by an increase of elastic fibers within dermis. The application of this simple scoring algorithm can be extended to the measurement of other physiological and pathological tissue conditions. For example, it can be used to determine the alteration of collagen/elastic fibers in a peritumoral region [62, 77] or to assess the fibrotic condition of the tissue [64]. In the example shown in this section, the SAAID algorithm is used to monitor the alteration of connective tissue that surrounds a basal cell carcinoma (BCC), as well as to score various altered dermal conditions, such as normal scars (NSs), keloid, and normal dermis. In the image shown in Figure 5.3a, BCC lesion, together with the perilesional dermis, was imaged using SHG microscopy (Figure 5.3a) and TPEF microscopy (Figure 5.3b) and merging the two images (Figure 5.3c). Tissue morphology highlighted by combined TPEF–SHG imaging demonstrated a good correlation with the corresponding histological image taken from an adjacent section of the same sample (Figure 5.3d). Within the images, three different regions with different architectural features are clearly distinguishable: the region with the tumor cells of BCC (B); the region delimiting the tumor, histologically known as tumor–stroma interface (S), and the surrounding connective tissue (D). BCC cells provided exclusively TPEF signal, while within the normal dermis both TPEF and SHG signals were found. A morphological modification was observed at the level of the tumor–stroma interface. In particular, a thin collagen layer emitting a strong SHG signal was observed corresponding to the tumor–stroma interface. On the other hand, no TPEF fluorescence was observed in this region. The morphological features of these three tissue regions were evaluated using the SAAID scoring method, finding that both BCC and the surrounding dermis have a negative SAAID value and the tumor–stroma interface a positive SAAID value, because of the high collagen SHG contribution and the reduced TPEF signal. The average SAAID values for the three representative regions are shown in the histogram in Figure 5.3e for the examined sample.

Figure 5.3 Transversal optically sectioned ex vivo Basal Cell Carcinoma (BCC) sample imaged using (a) SHG microscopy, (b) TPEF microscopy, (c) merging the SHG and the TPEF image, and (d) the corresponding histological image. Field of view: 800 μm × 800 μm. Scale bars: 80 μm. BCC (B), tumor–stroma interface (S), and surrounding connective tissue (D) are highlighted by the corresponding letters. The SAAID score, calculated for the three highlighted regions (dashed curves), is plotted in the bar graph in (e).
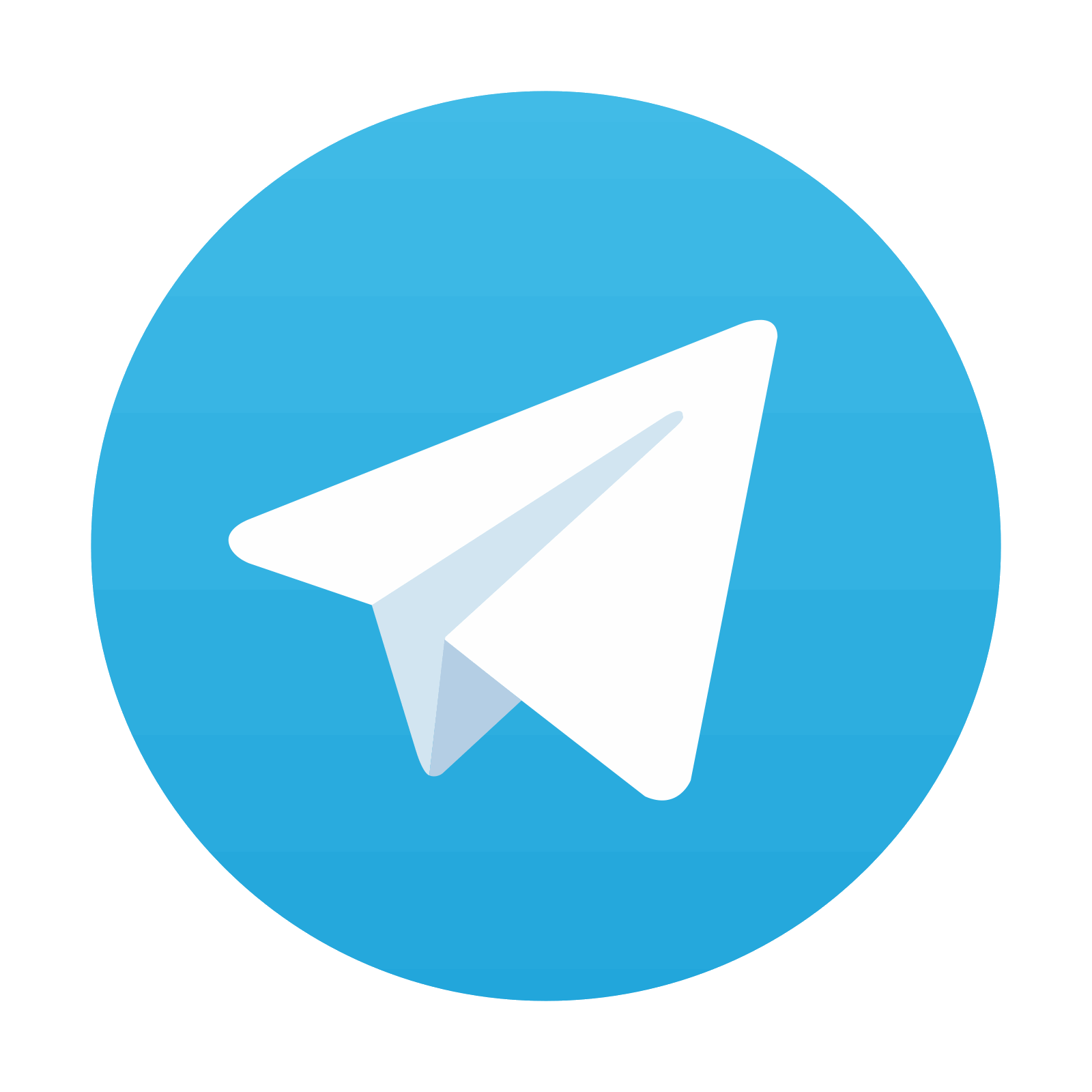
Stay updated, free articles. Join our Telegram channel
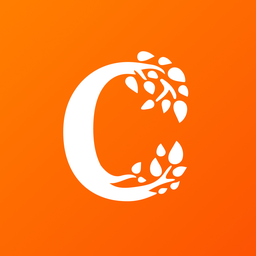
Full access? Get Clinical Tree
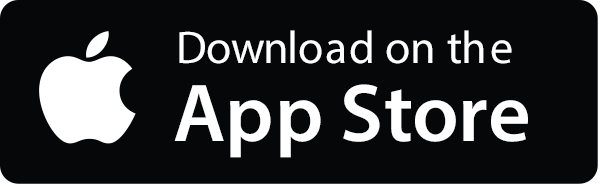
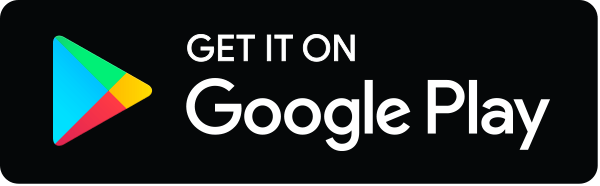