Fig. 17.1
(a) Kaplan-Meier survival plots for baseline DCE-MRI parameters in breast cancer patients scheduled to undergo neoadjuvant chemotherapy. Left: overall survival plot for the relative signal enhancement at 30 s after enhancement onset (EI30). Right: disease-free survival plot for the area under the enhancement curve (AUC) (Reprinted from [9], Copyright 2009, with permission from Elsevier). (b) Left: axial maximum intensity projection of a DCE-MR image of a breast with a palpable mass (P) and a nonpalpable, incident lesion (I). Center: the palpable mass (benign fibroadenoma) displayed a persistent-type signal intensity-time curve. Right: the incident lesion (invasive ductal carcinoma) displayed a washout-type signal intensity-time curve. (c) K trans maps from a patient with a colorectal liver metastasis showing decreased perfusion, vascularization, and/or vessel permeability after bevacizumab treatment (Reprinted by permission from Macmillan Publishers Ltd.: Nature Reviews Clinical Oncology [15], Copyright 2012). (d) Conventional T 2-weighted (T 2 w) images (left) and DSC-MRI-derived rCBV (center) and rCBF maps (right) from a patient with grade IV glioblastoma (top) and a patient with grade II astrocytoma (bottom). The differences in rCBV and rCBF between the high- and low-grade gliomas are readily apparent (Reprinted from [20], Copyright 2005, with permission from Elsevier)
The shape of DCE-MRI signal intensity-time curves has been shown to have diagnostic value in breast cancer [11]. Enhancement curves are typically classified as one of three types—persistent (continuous enhancement over time), plateau (enhancement reaches a plateau), or washout (initial enhancement followed by signal decrease). Most benign breast tumors (83.0%) displayed persistent enhancement, whereas malignant lesions were characterized by plateau- (33.6%) or washout-type (57.4%) curves (Fig. 17.1b) [11]. Such qualitative classification of enhancement curves may still be the most common form of DCE-MRI analysis, but efforts have been made to automate this classification to eliminate intra- and interobserver variability [12, 13].
Quantitative parameters are desirable in the context of treatment monitoring and drug trials as they enable better assessment of longitudinal changes and comparison between different centers and studies. It has been recommended that K trans or the initial area under the contrast agent concentration-time curve (IAUC) should be used as primary end points in early-phase cancer drug trials [14]. Anti-angiogenic therapies are expected to decrease vascularization, perfusion, and/or vessel permeability, which would lead to decreases in K trans and IAUC. Many clinical and preclinical studies have utilized DCE-MRI for monitoring response to various anti-angiogenic and vascular disrupting agents, with most reporting significant reductions in K trans and IAUC (Fig. 17.1c) [15, 16]. However, some studies showed no significant change, which may simply indicate drug resistance or point to the complexity of the therapeutic mechanisms of action and of the physiological meanings of the DCE-MRI readouts. It is still not fully clear how anti-vascular agents work, and this uncertainty is also present in the interpretation of K trans and IAUC, which are dependent on several factors (perfusion, vessel permeability, and surface area) that may change and affect these parameters in different ways after treatment. There are other, more generalized PK models that provide separate estimates of blood flow and the vessel permeability surface area product, which are reviewed by Sourbron and Buckley [17]. But they are also more complex and computationally expensive, require higher temporal resolution, and have not been widely adopted in clinical practice.
Susceptibility contrast MRI utilizes (super)paramagnetic contrast agents to measure vascular function and morphology. The difference in the magnetic susceptibilities of the i.v.-administered contrast agent and biological tissue locally enhances transverse relaxation rates (R 2 (*)) in and around blood vessels. Pre- and post-contrast images are acquired to measure the increase in relaxation (ΔR 2 (*)), which is dependent on contrast agent concentration and distribution.
In the clinic, dynamic susceptibility contrast (DSC)-MRI is used to measure perfusion, primarily in the brain. A series of pre- and post-contrast T 2 w or T 2*w images are acquired with high temporal resolution to capture the first pass of a GBCA bolus through the vasculature. Relative cerebral blood volume (rCBV) can be estimated from the signal intensity-time curve [18]; an AIF in addition to complex mathematics is required for quantification of absolute CBV and cerebral blood flow (CBF) [19]. DSC-MRI has been shown to be able to distinguish between high- and low-grade gliomas, with the high-grade lesions having significantly higher rCBV and rCBF (Fig. 17.1d) [20]. Similarly, Schmainda et al. showed that rCBV is predictive of OS in patients with recurrent high-grade glioma who received bevacizumab treatment, with OS being significantly longer if the rCBV of the lesion was below a certain threshold [21]. DSC-MRI is also sensitive to therapeutic response, but differentiating tumor progression from pseudoprogression and, in the case of anti-angiogenic therapy, response from pseudoresponse can be challenging [22, 23].
Intravascular contrast agents with long circulation times such as ultrasmall superparamagnetic iron oxide (USPIO) nanoparticles are used preclinically for steady-state susceptibility contrast (SSC)-MRI (i.e., vessel size imaging). Pre- and post-contrast spin and gradient echo images are acquired to measure steady-state ΔR 2 and ΔR 2*, respectively. These can be used to calculate various parameters that estimate mean vessel density [24], fractional blood volume, and mean vessel diameter [25]. SSC-MRI parameters have been shown to correlate with vascular measurements from histology [26] and high-resolution micro-CT [27, 28]. A review by Emblem et al. discusses the potential use of vessel size imaging parameters as clinical biomarkers of treatment response [29].
To summarize, there are multiple MRI techniques that are widely used preclinically and in clinical oncology for characterization of the vascular phenotype, diagnosis, and treatment evaluation. But a better understanding of the underlying biophysics that affect the MRI measurements and of the mechanisms of action of anti-angiogenic drugs and other therapies is needed for the development of clinically validated MRI-based biomarkers of tumor angiogenesis.
Imaging Tissue Cellularity and Microstructure
Diffusion-weighted MRI (DWI) is currently one of the fastest developing MRI-based techniques in oncology. DWI allows the mapping of water diffusion due to the Brownian motion of water molecules in vivo. The water diffusion is measured indirectly as a signal loss induced by diffusion-sensitizing magnetic field gradients applied during the MRI pulse sequence. The diffusion can be quantitatively assessed by calculating the apparent diffusion coefficient (ADC) value. This assessment is done by acquiring several (at least two) images with different diffusion sensitization, from which the ADC value can be derived by exponential fitting. High ADC values indicate relatively free diffusion, while low indicate restricted diffusion. The distribution of ADC values is commonly illustrated in parametric maps (Fig. 17.2a).


Fig. 17.2
(a) Pimonidazole staining of luminal-like breast cancer xenografts (top left) demonstrates that these tumors are more hypoxic than basal-like breast cancer xenografts (bottom left). ADC values were significantly lower in the luminal-like (top right) compared to the basal-like tumors (bottom right), which demonstrates the inverse correlation between hypoxia and ADC (Reprinted with permission from [48], Copyright 2011 Wiley Periodicals, Inc.). (b) Autoradiography (top left) demonstrates high accumulation of 18F-MISO (dark areas) in regions positive for pimonidazole (top right, green areas), whereas minimal tracer accumulation is seen in areas negative for pimonidazole. The difference between these tissues is difficult to recognize in an H&E section of the tumor (bottom left). Accumulation of 18F-MISO in hypoxic regions is also seen using in vivo PET imaging (bottom right) (Reprinted from [111], Copyright 2009, with permission from Elsevier)
The contrast in DWI arises from the different compositions of biological tissue, such as cell membranes, macromolecules, fibers, or other tissue components, all of which restrict water diffusion. Diffusion is also affected by water exchange between intracellular and extracellular compartments, the shape of the extracellular space, and tissue cellularity. Diffusion patterns can therefore reveal microscopic details about the tissue architecture. Due to this complex mixture of contributions to the measured DWI signal, the complete biophysical interpretation is still not fully clear.
Several studies have been performed to better understand the association between the microenvironment and the obtained diffusion properties. Importantly, tumor tissue is usually characterized by low water diffusivity, which is most likely related to higher cellular density [30] and proliferation [31]. Low ADC values have also been associated with high hypoxic fraction (Fig. 17.2a), interstitial hypertension, and elevated metastatic propensity in melanoma xenografts [30].
Epithelial-to-mesenchymal transition (EMT) is important for tumor metastasis. It has been proposed that an increasing ADC value can be detected in tumor cells undergoing EMT [32]. Water diffusion is altered during the transition between epithelial and mesenchymal phenotypes due to changes in cell-cell contact and the volume of extracellular space. Subcutaneous xenograft tumors with epithelial-like phenotypes showed significantly lower ADC values compared to those with mesenchymal-like phenotypes.
Exploiting the diffusion properties by histogram analysis of ADC values across a tumor volume enables a better description of intratumoral heterogeneity compared to using mean or median ADC. Such analyses have, for example, been utilized to differentiate gliomas [33]. Moreover, histogram analysis of ADC values of glioma contributed to distinguish between isocitrate dehydrogenase gene mutation-positive and mutation-negative high-grade gliomas, which could be relevant for patient management since the mutation-positive patients have a favorable prognosis [34].
Restriction spectrum imaging (RSI) is a new extension of the DWI methodology [35]. Restricted diffusion is a term used to describe the trapping of water molecules within an enclosed compartment, for example, as defined by the cell plasma membrane. RSI requires the use of high diffusion sensitization in addition to directionality and enables quantitative estimates of tissue microstructure based on modeling of tissue properties such as cell size, density, and orientation as a function of diffusion sensitization. The calculated cellularity index, an in vivo measure of spherically restricted water, has shown promising clinical results in improving the tumor conspicuity of high-grade brain tumors [36], capturing the treatment response of anti-angiogenic treatment [37], and improving visualization of white matter pathways through regions of peritumoral edema [38]. More recently, the RSI-derived cellularity index was found to be associated with the aggressiveness of prostate cancer (Gleason score) [39] and significantly contributed to prostate cancer staging based on accurate detection of extraprostatic extension of the tumor [40].
Anticancer treatment will change structural features of the tumor tissues and cells. Treatment-induced cell death is usually reflected by increased ADC values, due to loss of cell membrane integrity and decreased cell density caused by both necrosis and apoptosis, and this may be observed prior to any significant reduction of the tumor volume [41]. Increased diffusion due to treatment response has been shown after standard cytotoxic treatment [42, 43], targeted treatment [43], anti-angiogenic treatment [44], and radiation therapy [45]. A comprehensive review of DWI in oncologic applications can be found in [46].
The relationship between diffusion and the tumor stroma has been investigated in breast cancer [47], where ADC values were negatively correlated with tumor/stroma ratio, most likely because stroma-poor tumors have higher cellularity. However, diffusion was observed to be lower in collagen-dominant stroma types compared to fibroblast- or lymphocyte- dominant types [47]. This is in agreement with findings in patient-derived luminal-like breast cancer xenografts, where a low ADC value was attributed to the high content of collagen and fibronectin in the stroma [48–50]. A recent work in prostate cancer also demonstrates that ADC correlates with the tumor tissue composition, and a positive association between ADC and the volume of the luminal space was identified [51].
An interesting extension of DWI is diffusion tensor imaging (DTI) , which adds information about tissue microstructure by addressing diffusion direction. In DTI, diffusion-sensitizing gradients are applied in many (at least six) different directions, and the diffusion profile is fitted to a tensor model. The tensor model assumes that one dominant direction of diffusion is present and that the diffusion anisotropy can be described by an ellipsoidal symmetry [52]. DTI has been suggested as a new approach for detection of breast cancer based on tracking the mammary architectural elements [53]. The breast’s fibroglandular tissue is orientated along tubular ducts and ligaments, and the diffusion properties of the mammary fibroglandular tissue change during malignant transformation. The sensitivity of DTI to detect breast cancer was found to be high, particularly in dense breasts. A challenge in the management of breast cancer is the detection of early response to therapy. DTI could potentially aid in this, as changes in diffusion anisotropy are expected to reflect changes in tissue structure induced by neoadjuvant therapy. Fractional anisotropy (FA) describes the degree of anisotropy of the diffusion process. FA and ADC were found to correlate with collagen fiber density in breast cancer xenografts [54]. The hypoxic regions of the same xenografts contained lower density of collagen fibers and simultaneously exhibited lower FA and ADC, suggesting that ADC and FA could serve as clinically relevant, noninvasive markers of fiber density as well as hypoxia.
Intravoxel incoherent motion (IVIM) imaging is another advanced DWI approach that was first described nearly three decades ago but is now gaining huge interest in oncology [55]. This technique takes into account the fact that the motion of water molecules contributing to the diffusion signal not only arises from extra- and intracellular diffusion but also from intravascular blood flow (perfusion). This is especially apparent for images acquired with low diffusion sensitization [56]. IVIM allows for the separation of motion of water molecules due to microcirculation from motion due to diffusion, which is promising for response measurement in treatment studies targeting both vasculature and cell proliferation [57]. Importantly, this reflection of tissue diffusivity and microcapillary perfusion is obtained without contrast agent injection.
In conclusion, DWI is now frequently used as one of the sequences in multiparametric MRI for preclinical and clinical oncological applications. The DWI signal, and the derived ADC value, largely depends on the tissue cellularity. To establish ADC as a robust biomarker, standardization of the DWI acquisition (how to apply the diffusion sensitization) and subsequent post-processing and analysis is necessary [58]. Importantly, preclinical studies have shown that absolute ADC values are comparable between sites and equipment, provided standardized protocols are employed [59]. Advanced extensions of DWI, such as DTI, RSI, and IVIM, broaden the applicability of the methodology. DWI offers promising biomarkers for both cancer detection and evaluation of treatment response and opens a window for in vivo, noninvasive characterization of the tumor microenvironment.
Investigating Cancer Metabolism
During cancer progression, molecular changes are associated with metabolic reprogramming [60, 61], which is a hallmark of cancer [62]. Metabolic changes can be measured by MRS at the molecular level, providing insights into the causes of altered metabolism in oncogenesis. This technique can be performed on current clinical MR systems in vivo or within cell/tissue extracts and intact tissue samples in laboratory settings using high-resolution MRS [63, 64].
MRS exploits the fact that the Larmor frequency is different for every nuclide and also depends on the magnetic field strength. Different nuclei experience slightly different magnetic fields depending on their molecular environment and chemical shielding and therefore precess at slightly different frequencies. These resonance offsets can be described on a field-independent dimensionless scale called chemical shift (δ), which is expressed in parts per million (ppm). Individual molecular properties can be characterized by a single or multiple resonances in MR frequency spectra, thereby allowing detection and quantification of the relative concentrations of different metabolites and other molecules via MRS.
One of the most common metabolic anomalies observed in cancer is the Warburg effect. Partly due to hypoxia and partly due to direct metabolic regulation through oncogenic signaling, most cancer cells exhibit high glycolytic activity and convert a substantial fraction of their glucose to lactate even in the presence of adequate oxygen levels [65]. The lactate dehydrogenase (LDH) enzyme catalyzes the reversible conversion of pyruvate to lactate. LDH expression and high tumor lactate are required for the progression of many tumors. MRS-measured lactate concentration can be a good indicator of the metabolic adaptation in cancer cells, and many studies have revealed its correlation with prognosis, treatment efficacy, and clinical outcome in a variety of human cancers [66]. Some other commonly measured metabolites in 1H MRS include choline-containing compounds, lipids, N-acetyl aspartate, creatine, glutamate, glutamine, GABA, myoinositol, citrate, and 2-hydroxyglutarate.
Altered membrane choline phospholipid metabolism is associated with malignancies, oncogenesis, and tumor progression [67]. Changes in choline-containing metabolite concentrations have been shown to be potential early biomarkers of targeted antitumor therapies. Increased levels of choline-containing metabolites, referred to as total choline (tCho), in cancer cells has been interpreted to be associated with cancer cells’ demands for increased proliferation, upregulation of choline kinase activity, and oncogenic cell signaling such as overactivity of PI3K signaling [67]. 1H MRS can detect tCho noninvasively on clinically available MR scanners. 31P MRS is another useful tool for noninvasive investigation of phospholipid metabolism in vivo. Compared to 1H MRS, this technique is less prone to water and lipid contamination; however, it is less sensitive and requires an additional dedicated phosphorus coil. A number of key metabolites involved in phospholipid metabolism are detected using this method, such as phosphocholine (PCho), phosphoethanolamine (PE), glycerophosphocholine (GPC), and glycerophosphoethanolamine (GPE) (Fig. 17.3a–d). Other potential applications of 31P MRS include the evaluation of high-energy phosphates: phosphocreatine (PCr), adenosine triphosphate (ATP), and adenosine diphosphate (ADP) (Fig. 17.3d).


Fig. 17.3
(a–c) 31P MRSI voxels (27 mm3 nominal resolution) overlaid on orthogonal T2-weighted MR images of a tumor-bearing mouse brain. (d) Corresponding 31P MR spectrum of the voxel outlined in blue from the 3D 31P MRSI data. The assigned peaks in (d) are (from left to right) PE phosphoethanolamine, PC phosphocholine, Pi inorganic phosphate, GPE glycerophosphoethanolamine, GPC glycerophosphocholine, PCr phosphocreatine, and ATP adenosine triphosphates (Reprinted with permission from [112]). (e–i) Axial images of the right front leg of a canine cancer patient with liposarcoma. Note the high concentration of 18F-FDG in the muscle (arrow, panel F, 18F-FDG-PET overlaid on 1H-MRI) and of 13C-pyruvate in the large vessels (arrow, panel H, 13C-pyruvate CSI + 1H-MRI), as well as increased level of 13C-lactate in the tumor (arrow, panel I, 13C-lactate CSI + 1H-MRI). CSI chemical shift imaging (Reprinted with permission from [76])
With 1.1% natural abundance of the 13C isotope, 13C MRS of endogenous metabolites is less sensitive than 1H MRS. However, exogenous 13C MRS is feasible following administration of a 13C-labeled substrate, including 13C-labeled glucose, and subsequent incorporation of the 13C label from the exogenous substrate into other molecules. For instance, glycolytic rates can be measured by investigating the uptake and metabolism of [13C]-glucose in vivo. However, the utility of 13C MRS is limited due to its relatively low sensitivity and the fact that most commercial MR systems are only capable of 1H MRS. One of the most important innovations in recent years has been the development of dynamic nuclear polarization (DNP, or “hyperpolarization”) of 13C-labeled metabolic substrates, enhancing the sensitivity of the 13C MRS experiment dramatically (> 10,000-fold higher than non-hyperpolarized 13C) [68]. This improvement allows the analysis of several metabolic pathways and metabolic fluxes through select enzyme-catalyzed steps. Due to its excellent polarization properties, rapid transport into important metabolic pathways, and longer relaxation time, [1-13C]pyruvate has been the most widely studied substrate to date. In addition to indicating the presence of the Warburg effect in tumors, [1-13C]pyruvate has been employed to investigate the response to anticancer therapies by noninvasively evaluating real-time flux of pyruvate to lactate and LDH activity [69, 70]. [1,4-13C2]fumarate has been used to investigate cell necrosis and treatment response in tumors [71]. Hyperpolarized [1-13C]pyruvate and [1,4-13C2]fumarate have also been used for detection of early changes in tumor metabolism following administration of a vascular disrupting agent [72].
Positron emission tomography (PET) is a sensitive and quantitative method for measuring the uptake and trapping of different radiolabeled PET substrates, such as 18F-fluorodeoxyglucose (18F-FDG), a radioactive form of glucose. This technique allows noninvasive molecular imaging of cancer cell metabolism, heterogeneity, and metastases in systems ranging from advanced tumor models to patients in the clinical setting. When combined with computed tomography (CT), PET/CT provides both anatomical localization and functional information. There is a wide range of novel and established PET radiotracers, which can be used to investigate various aspects of cancer, including carbohydrate, amino acid, and fatty acid metabolism. 18F-FDG-PET by far is the most successful tracer in in vivo cancer studies. The reduction in 18F-FDG uptake has been used to detect treatment response in some cancer subtypes [73].
Integration of 18F-FDG-PET and MRS may potentially increase the sensitivity and accuracy in tumor localization [74] and specificity of tumor detection [75, 76] (Fig. 17.3e–i). The increased availability of clinical PET/MR scanners has recently raised the interest in simultaneous DNP-MRS and PET imaging [76, 77]. PET provides relatively higher sensitivity than DNP-MRS, detecting in the range of nano- to picomolar compared to the millimolar range sensitivity of MRS. Due to low sensitivity, the concentrations of DNP substrates that have to be administered may exceed that of physiologic levels, which may perturb normal metabolism. The short half-life (in tens of seconds) is another notable limitation of DNP-MRS, which calls for improvement in fast MRS data acquisition. But unlike PET, DNP-MRS does not employ ionizing radiation and can detect injected substrate and its metabolic products simultaneously. The latter enables the observation of both the uptake of the targeted molecule and its downstream metabolic products.
Multimodal imaging techniques have provided novel opportunities for cancer treatment by providing comprehensive cancer metabolomic information. In clinical cancer management, there has been a great tendency toward personalized therapies—including targeting specific metabolic pathways, enzymes, and/or oncogenes—and away from aggressive or cytotoxic treatments. Integration of anatomical information of MRI and metabolic information provided by multivoxel MRS imaging (MRSI) or PET can significantly improve the assessment of cancer location, extent, aggressiveness, and response to treatment.
Imaging Tumor Hypoxia
Hypoxia can be defined as subnormal levels of oxygen in tissues and is a frequent phenomenon in solid tumors. A functional mismatch between cell proliferation and vascularization often leads to poor oxygenation of tumor regions—either because the cancer cells are located so far from the nearest blood vessel that oxygen supply through diffusion is insufficient or because the tumor vasculature is dysfunctional and cannot provide oxygen to meet the demands of the surrounding tumor tissue [78, 79].
During the life span of a tumor, there will be both temporal and spatial variations in the degree of hypoxia. This will in turn induce adaptive changes in the biology of the cancer cells. These changes are predominantly mediated through the hypoxia-inducible factors (HIFs) and hypoxia response elements, which transcriptionally regulate genes that are relevant for cancer cell growth and disease development [80].
A well-documented clinical consequence of low partial oxygen pressure in tumors is resistance to radiotherapy caused by insufficient production of free oxygen radicals [81]. However, it has also been shown that hypoxia is associated with poor prognosis in several cancers [82]. Furthermore, it has been demonstrated that hypoxia promotes local invasion and metastatic dissemination of cancer cells [83, 84].
Since hypoxia is a driving force for cancer progression and since the outcome of radiotherapy is strongly associated with oxygenation of the target tissue, measuring the level of hypoxia in solid tumors has potential clinical implications. There is therefore significant interest in development of noninvasive imaging methods that can report on the degree of hypoxia in solid tumors, preferably with high spatial resolution. This can be achieved either through direct approaches using oxygen-level-sensitive contrast agents or through indirect approaches using functional proxy markers of hypoxia.
MRI cannot directly measure the partial pressure of oxygen in the tissue. Therefore, hypoxia has traditionally been imaged using indirect markers of oxygen concentration. Oxygen delivery and consumption depend on vascular perfusion and cellular density, which can be imaged using DCE-MRI and DW-MRI, respectively. While these methods do not provide information on actual tissue oxygenation, several reports describe relationships between standard DCE-MRI and DW-MRI readouts and hypoxia in preclinical model systems. For example, an inverse correlation between K trans and ADC and the fraction of hypoxic cells has been demonstrated in several experimental model systems (Fig. 17.2a) [30, 48, 85, 86]. However, this may represent an indirect association because hypoxia can arise from the imbalance between oxygen supply and demand created by high cellular density. Interestingly, these studies have also demonstrated associations between these functional MRI parameters, hypoxia, and metastatic potential, emphasizing the potential clinical value of imaging the hypoxic tumor microenvironment.
Using blood-oxygen-level-dependent (BOLD) MRI , the ratio of oxygenated to deoxygenated blood can be measured through differences in intrinsic magnetic susceptibility between oxy- and deoxyhemoglobin—the presence of paramagnetic deoxyhemoglobin will increase the R 2* relaxation rate of water protons. In cancer, it has been suggested that changes in R 2* in response to inhalation of hyperoxic gas can be used to identify hypoxic tumor fractions [87]. However, changes in R 2* are not directly proportional to changes in tissue oxygenation levels, and this technique has therefore not yet found clinical use [88, 89]. The same problem applies to R 1 relaxivity-based tissue-oxygen-level-dependent (TOLD) MRI , which recently has been suggested as a tool for mapping regional oxygenation in tumors [90]. The role of BOLD and TOLD MRI in assessment of tumor hypoxia therefore remains unclear.
The direct approach of assessing hypoxia is predominantly based on 2-nitroimidazole derivatives, a group of compounds that form covalent bonds with cellular macromolecules at oxygen levels below 10 mmHg pO2 [91]. Nitroimidazole adducts can be detected ex vivo using immunohistochemistry [92], but contrast agents for in vivo labeling and imaging have also been developed. Several fluorinated nitroimidazole derivatives have been tested clinically, using 19F MRS to detect accumulation of contrast agent in hypoxic tumor regions [93]. Recently, a gadolinium-labeled nitroimidazole contrast agent (GdDO3NI) was found to accumulate in poorly perfused regions of xenografted tumors, suggesting that T 1-based MRI of hypoxia may be a possibility [94]. However, efforts to develop hypoxia-targeted MRI contrast agents are hampered by the inherent low sensitivity of this imaging modality.
In contrast, PET imaging is highly suitable for quantitative imaging of contrast agents present in low concentrations in the tissue. Several PET probes, such as 18F-fluoromisonidazole [18F-MISO] and 18F-flortanidazole [18F-HX4], bind to viable hypoxic cells in vivo, thereby allowing direct imaging of hypoxia in cancer (Fig. 17.2b). Using quantitative readouts such as tumor/blood or tumor/muscle signal intensity ratios, it has been shown that 18F-MISO can accurately and reproducibly image regional insufficiencies in pO2 across a wide range of cancers [95, 96]. In several trials, pretreatment 18F-MISO uptake predicted the outcome of radiation therapy, demonstrating the value of noninvasive hypoxia assessment [97, 98]. The technique has been cross-validated against DCE-MRI, demonstrating that high 18F-MISO uptake correlates with low K trans. Based on the current clinical evidence, the FDA has granted an Investigational New Drug (IND) status for 18F-MISO.
In summary, the clinical implications of noninvasive assessment of tumor tissue oxygen levels are significant, predominantly due to its predictive value in radiotherapy. Currently, PET imaging with 18F-MISO is the most widely used imaging approach. As the BOLD and TOLD MRI techniques do not require the use of exogenous contrast, further understanding of how they reflect tumor tissue oxygenation would make them attractive for clinical use.
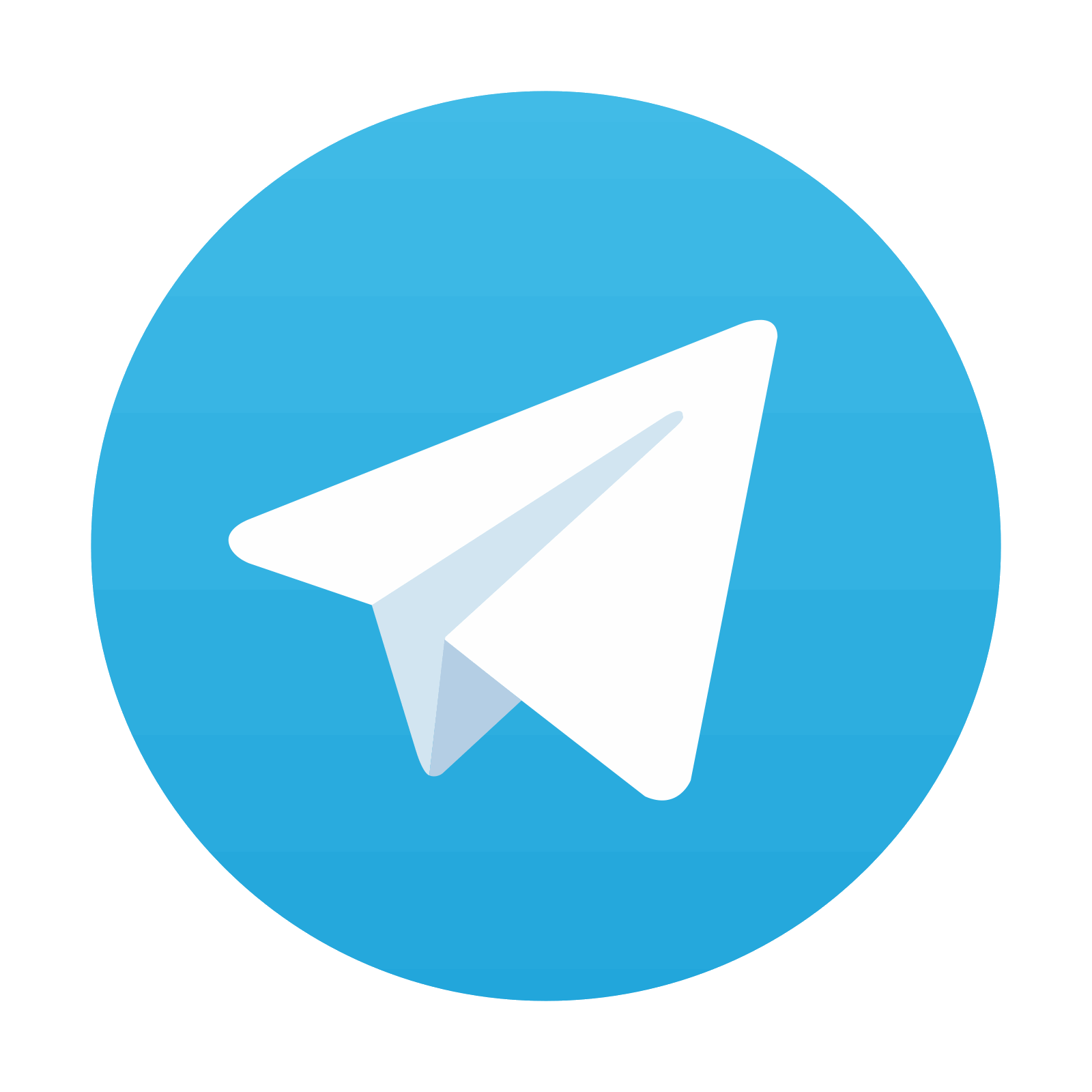
Stay updated, free articles. Join our Telegram channel
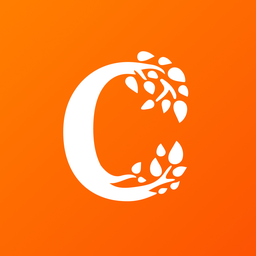
Full access? Get Clinical Tree
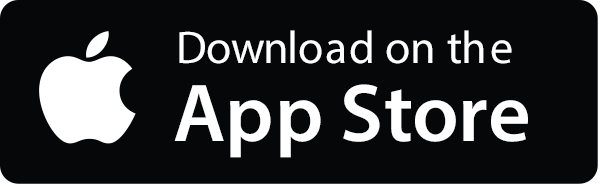
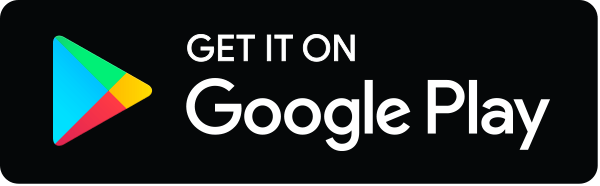