Fig. 2.1
Phenotypic and molecular heterogeneity of endothelium at different metastatic sites. Although the endothelium is a common barrier encountered by tumor cells at all metastatic sites, tissue-specific heterogeneity means the barrier encountered varies between tissues. (a) Examples of the differences encountered between continuous capillaries (found in the brain, strongest barrier), fenestrated (found in the kidney), and sinusoidal (found in the liver, slightest barrier) are provided for comparison. In particular, differences include the type of junctions between endothelial cells, presence/absence of a diaphragm, fenestrations and intercellular clefts, basement membrane density, and the associated resident tissue cell types within each perivascular microenvironment. Furthermore, the phenotypic and molecular heterogeneity between resident endothelial cells means that mechanisms coopted by tumor cells to extravasate at various metastatic sites are specialized. (b) This panel illustrates how tumor cells may interact with the endothelium encountered within the brain or liver during extravasation. (b( i )) In the brain, tumor cells express ST6GALNAC5, a sialyltransferase [47]. It is thought ST6GALNAC5 modifies cell adhesion molecules expressed by brain endothelial cells through sialylation, which subsequently permits the tumor cell to selectively interact with the brain endothelium and then transmigrate through the blood-brain barrier. (b( ii )) In the liver, tumor cell entry into the hepatic sinusoids triggers a rapid pro-inflammatory cascade (1) activating local Kupffer cells which secrete TNFα and IL-1β (2) This increases E-selectin, ICAM-1, and VCAM-1 expression on LSECs (3) facilitating tumor cell adhesion (4) and subsequent transmigration through the LSEC barrier [139, 140]
Although overt phenotypic disparities between microvascular ECs located in different tissues have long been observed, the molecular profiles underlying observed phenotypes are only beginning to be elucidated. A very insightful study aimed to determine the molecular signature (in terms of the transcription factors, adhesion molecules, metabolic profiles, and surface receptors) expressed by tissue-specific (liver, bone marrow, kidney, heart, lung, brain, muscle, spleen, testis) microvascular ECs during organ homeostasis and regeneration [7]. Importantly, similarity between molecular phenotypes closely reflected shared phenotypes of tissue-specific endothelia. For example, liver microvascular endothelium was found to be most similar to the spleen and least similar to the lung, whereas brain microvascular endothelium was most similar to the heart and dissimilar to the bone marrow. Additionally, as with seminal studies performed decades ago showing epithelial specification by stroma [8] (recently reviewed by [9]), stroma (and other microenvironmental cues) also confers tissue-specific profiles/properties to implanted “generic” ECs [7].
In the context of the discussion that follows, it is important to consider endothelial heterogeneity and the likely differences in a tissue’s perivascular microenvironment when contemplating how different phenotypes of perivascular tumor cells emerge. Even when the end result is identical, the factors that guide the said phenotype from tissue to tissue are likely to differ.
The Endothelium as a Barrier to Metastasis
The metastatic cascade comprises a number of steps that a tumor cell must accomplish to establish a metastatic lesion at a secondary tissue site. Successful metastatic progression requires that tumors cells escape from a primary site, enter the lymphatic system or bloodstream (intravasation), exit from the circulation (extravasation), survive within a non-native tissue, and colonize secondary tissues. Thus, it is clear that endothelium is a physical barrier that tumor cells must successfully interact with and navigate in order to progress through the cascade (Figs. 2.1b and 2.2). A number of examples of precisely how tumor cells and ECs interact at the molecular level, particularly within the context of intravasation and extravasation, are provided below. Broadly, for tumor-EC interactions to occur, both parties must express complementary ligand-receptor pairs. However, the complexity of potential molecular interactions becomes evident when one considers the array of primary tumor cell origins and the heterogeneity of endothelium across tissues.


Fig. 2.2
The endothelium has direct contact with tumor cells during all steps of the metastatic cascade. (a) Although this example illustrates a patient with breast cancer and dissemination to the bone marrow, the steps involved in metastatic dissemination are similar for all types of primary cancer and secondary sites. The top right panel illustrates a closeup view of the cellular interactions involved in the initial steps of the metastatic cascade up until intravasation. Namely, these are (1) epithelial tumor cell escape from neoplastic tissue or tumor, (2) invasion through the basement membrane, and (3) intravasation and escape into either the circulatory or lymphatic system following direct molecular interaction with endothelial cells. Note the paracellular or transcellular route that the tumor cells can take during the transendothelial migration process. (b) During extravasation, tumor cells directly interact with the luminal face of the endothelium and can either undergo a transcellular or paracellular route. There are four possible outcomes for the tumor cell once it has encountered a new tissue microenvironment at a secondary tissue site, which is a perivascular locale: (1) the tumor cell encounters an incompatible microenvironment and subsequently dies. (2) The tumor cell encounters a perivascular microenvironment containing stable microvascular endothelium and enters into a state of cellular dormancy. (3) The tumor cell slowly divides, driven primarily by cell autonomous cues. (4) The tumor cell encounters a perivascular microenvironment in which the endothelium is undergoing active remodeling; this supports tumor cell growth and is one of the initial steps leading to metastatic colonization
Tumor Cell Intravasation
Transendothelial migration is a process used by cancer cells use to traverse the endothelial barrier when entering or exiting the circulation. Typically, vessels found in a tumor mass have been recently remodeled via angiogenesis—i.e., new blood vessels formed from preexisting blood vessels. This indicates that blood vessels in tumors are often immature and leaky due to weak cell–cell junctions, so tumor cells can more easily enter the circulation. Tumor cells, in particular metastatic breast cancer cells, have been found to transmigrate either through EC junctions (paracellular intravasation) or by penetrating the actual EC itself (transcellular intravasation) [10, 11] (Fig. 2.2a).
In paracellular intravasation , varied molecular interactions between tumor cells and ECs lead to the separation of cell junctions between ECs, providing a “path” for the tumor cell to enter the circulation. A number of signaling mechanisms are implicated in mediating paracellular intravasation. In colon cancer, amino-terminal enhancer of split (Aes) expression is lost at the invasive front of the primary tumor in vivo [12]. In culture, it was demonstrated that loss of Aes expression in colon cancer cells leads to activation of the Notch signaling pathway, with Jagged1 ligand expressed by ECs and Notch1 receptor by the cancer cells. Aes typically keeps the Notch pathway transcriptionally repressed; however when Aes is lost, the Notch pathway becomes transcriptionally active and promotes transendothelial migration [12].
A second molecular mechanism that cancer cells may exploit in order to intravasate involves upregulated expression of ADAM12 (a disintegrin and metalloproteinase) on the vasculature during breast cancer progression [13]. ADAM12 is a protein capable of converting membrane-anchored ligands and cytokines into soluble, active forms. This is known as “ectodomain shedding.” ADAM12 releases active ligands and growth factors such as Sonic Hedgehog [14], Delta-like 1 [15], heparin binding-epidermal growth factor (HB-EGF) [16], and endothelial-specific proteins such as Flk-1, Tie2, vascular cell adhesion molecule (VCAM)-1, and VE-cadherin [13]. Importantly, VE-cadherin is involved in maintaining adherens junctions: protein complexes found at cell–cell junctions. Demonstration that ADAM12 enhances endothelial-derived shedding of VE-cadherin suggests that it facilitates breakdown of EC–EC junctions and is another molecular mechanism cancer cells take advantage of in order to intravasate.
Endothelial and tumor cells also communicate with other cells within their local microenvironment during intravasation. Using two-photon microscopy, researchers identified a particular anatomical structure they called a “tumor microenvironment of metastasis (TMEM)” that formed prior to intravasation of mammary tumor cells in mice [17–19]. This anatomical structure contains mammary tumor cells expressing high levels of mammalian enabled homologue (MENAINV; an invasion-specific spliced variant), in direct contact with both macrophages and ECs [17]. Alternative splicing of MENA is associated with increased breast tumor invasiveness through increased sensitivity to epidermal growth factor (EGF) [20–22]. Macrophages supply EGF to the MENAINV high tumor cells. This subsequently enhances the number of tumor cells that intravasate into the circulation [23]. Notably, other mechanisms exist whereby cancer–EC molecular interactions inevitably lead to rearrangement of cell junctions between ECs, allowing cancer cell passage into circulation; the reader is directed to this review for more examples [24]. Crucially, the main concept is that direct molecular interactions and cross talk occurring between cancer cells and endothelium enable intravasation.
Less is known about transcellular intravasation (Fig. 2.2a), which is also termed “emperipolesis”—meaning the presence of an intact cell (tumor cell) within the cytoplasm of another cell (EC). Tumor cell transcellular intravasation shares many parallels with leukocyte transcellular intravasation [25–28], with most mechanistic insight for tumor cell transcellular intravasation coming from studying endothelial–leukocyte dynamics. Nevertheless, it has been shown that breast tumor cells can be internalized by ECs through a molecular mechanism mediated by myosin light chain kinase (MLCK) . Activation of MLCK by Ca2+-calmodulin allows phosphorylation of MLC. The result is contraction of an actomyosin protein complex and creation of a pore-like structure in the EC due to the rearrangement of the endothelial cytoskeleton, which can subsequently envelop the tumor cell [11, 29].
Cancer cell dissemination via the lymphatics is also important in metastatic progression, yet surprisingly little is known about how tumor cells enter the lymph system. Although junctions formed by lymphatic ECs are composed of proteins typically found in tight and adherens junctions, unique junctions are formed. The lymphatic endothelium located at the beginnings of the lymphatics is linked together by “button”-like junctions, providing a leakiness to this section of the lymphatic vessel that allows direct fluid flow without disassociation of cell–cell junctions [30]. Incidentally, this proximal portion of the lymphatics is also where the majority of leukocytes enter the vessels. Conversely, the collecting lymphatics contain “zipper”-like junctions, which provide a continuous barrier between cells without openings [30].
Due to the architecture of the proximal lymphatics, it was long assumed that tumor cell entry into the lymphatic channels was a passive process. However, the molecular mechanisms mediating active tumor cell entry into the lymphatics are currently under investigation. The enzyme 15-lipoxygenase-1, which catalyzes the breakdown of arachidonic acid, is one such mediator of tumor cell intravasation into the lymphatics. Metabolites from this conversion disrupt lymphatic vessels by creating holes in the endothelium through which mammary carcinoma cells can penetrate via bulk invasion [31]. These holes are due to centrifugal migration of lymphatic ECs rather than apoptosis. Lymphatic ECs are seven times more prone to lipoxygenase activity than blood ECs, indicating a specific mechanism for lymphatic infiltration by tumor cells [31]. Similar endothelial disruptions have also been observed with melanoma cells cultured on lymphatic EC monolayers [32].
Additionally, it should be noted that the majority of studies examine intravasation in the context of a primary tumor mass, where tumor cells enter tumor-associated vessels with pathological phenotypes (discussed in section “Remodeling or Activated Endothelium and Metastatic Colonization”). Importantly, precise mechanisms of tumor cell intravasation are likely to differ in neoplastic tissue when tumor cells disseminate via relatively normal, physiological vessels.
Tumor Cell Extravasation
Similar to intravasation, tumor cells also negotiate the endothelial barrier in either a paracellular or transcellular manner upon exit (Fig. 2.2b). The main difference between intravasation and extravasation is that the tumor cell breaches the endothelial barrier from the opposite side in each process. This means that when a tumor cell approaches endothelium following dissociation from the primary tumor, it will be exposed to basally expressed proteins on the endothelium. Conversely, when the tumor cell approaches from the circulation, it will encounter apically expressed endothelial proteins. Therefore, a tumor cell must express cognate ligand and/or receptor proteins relevant to each process.
Once a tumor cell has entered the circulation, a combination of both mechanical and adhesive factors mediates its arrest within target organ capillaries [33]. Until the mid-2000s, it was still a popular idea that mechanical entrapment alone within smaller capillaries was sufficient to induce tumor cell arrest within microvessels. However, it has since been demonstrated that attachment and stable adhesion to the endothelium via different cell adhesion molecules is a requirement for transendothelial migration [33]. There are five major classes of cell adhesion molecules—selectins, cadherins, the immunoglobulin (IgG) superfamily, mucins, and integrins—that facilitate adhesion.
Initial Attachment: Selectins and Cadherins
Initial attachment of tumor cells to the endothelium is mediated by selectins (“selected lectins”—carbohydrate-recognizing proteins that bind sialylated carbohydrates, mediating heterotypic cell–cell adhesion) and cadherins (calcium-dependent adhesion—mediating homophilic cell–cell adhesion). Importantly, tumor cell attachment to endothelium via selectins can be initiated under high sheer stress, a scenario a tumor cell will more than likely encounter during dissemination via the circulation and subsequent arrival at a secondary site. Selectins expressed by ECs and relevant to metastasis include E- and P-selectin, which bind to a variety of ligands expressed by tumor cells. These include tetrasaccharide sialyl Lewis x (sLex) antigen, galectin-3-binding protein (LGALS3BP), Mucin 1, various glycoforms of CD44, P-selectin glycoprotein ligand 1 (PSGL1), and CD24 [24]. Cadherins , such as N-cadherin, can be expressed both by endothelial cells and tumor cells. Cadherin binding is mediated by self-interaction in the presence of calcium.
Stable Attachment: Integrins
Stable adhesion of tumor cells to the endothelium is mediated by integrins , a family of heterodimeric transmembrane receptors that transduce cell–cell and cell–extracellular matrix (ECM) interactions [34]. Integrins “integrate” outside cellular cues in order to dictate cellular responses such as cell adhesion, survival, migration, proliferation, and differentiation (reviewed by [35]). The capacity for integrins to command such a wide range of cellular outputs is in part due to the permutations available in the receptor repertoire. Integrins are composed of 18 α and 8 β subunits that can form at least 24 combinations of heterodimeric receptors, which can subsequently bind a number of ECM ligands [35]. Crucially, unlike selectin-mediated attachment, binding of tumor cells to integrins can only occur: (1) after initial selectin adhesion, due to the slow rate of integrin binding, or (2) under conditions of low sheer stress.
There are a number of relevant examples of how integrin binding between a tumor cell and endothelium dictates the resultant pattern of metastasis observed with different cancer types. For example, it has been shown that expression of the integrin ligand L1 cell adhesion molecule (L1CAM) by breast cancer tumor cells [36] mediates adhesion to its receptor αVβ3 integrin expressed on the luminal and abluminal face of lung microvascular endothelium [37]. This interaction mediates breast cancer cell homing and metastasis to the lungs, as well as spreading upon the basal surface of brain endothelium [38]. It has been proposed that the latter is a critical indicator of the capacity of a disseminated tumor cell (DTC) to colonize tissue [38].
Integrin expression by lymphatic endothelium also dictates metastatic outgrowth within the lymph node. Vascular endothelial growth factor (VEGF)-C production within the primary tumor microenvironment systemically promotes lymphangiogenesis—the growth of new lymphatic vessels from preexisting lymphatics [39]. VEGF-C-induced PI3Kα-mediated remodeling induces α4β1 integrin receptor expression on lymphatic endothelium [40]. In turn, this promotes colonization of the lymph node by VCAM-1+ metastatic tumor cells (such as gastric, breast, and renal carcinoma cells) [40].
Paracellular and Transcellular Extravasation
Once a tumor cell has stably adhered to the endothelium, the cell may exit by either a paracellular or transcellular route into the parenchyma (Fig. 2.2b). Currently, which route cancer cells prefer in vivo is unknown. In culture, the majority of cells take a paracellular path [41]. Few ligand-receptor pairs that mediate extravasation have been identified. However, one of these pairs is the interaction of endothelial-expressed CD31 (also known as platelet endothelial cell adhesion molecule (PECAM)-1, localized to EC–EC cell junctions) with tumor cell αvβ3 integrin [42]. Blocking αvβ3 integrin via antibody or RNAi prevents transendothelial migration, even though tumor cell adhesion to the luminal endothelial surface is unaffected [43]. Therefore, CD31/αvβ3 ligand-receptor pairing is a mechanism that specifically mediates extravasation.
Furthermore, the extravasation process can differ significantly in the time required for a tumor cell to traverse the endothelium, dependent upon what vascular bed the tumor cell is navigating. Tumor cells take much longer to extravasate into the brain than other organs. For example, breast and lung cancer cells take days to extravasate into the brain [44, 45], whereas lung cancer cells can extravasate into the liver in a matter of hours [45]. Breast tumor cells that successfully form macrometastases within the brain of mice were found to extravasate by day 3, although cells were able to extravasate until day 14 postinjection [46]. Some of the specific mechanisms tumor cells use in order to infiltrate the blood–brain barrier (BBB) are discussed below.
Breaching the Blood–Brain Barrier
It is important to recognize that tumor cells must navigate distinct endothelial barriers depending on the organ (and likely the part of the organ) into which they extravasate. As an example, navigating the brain’s microvascular endothelium presents a host of unique challenges. Brain capillaries are encapsulated by basement membrane, tight junctions, and astrocyte end feet, requiring tumor cells to co-opt more advanced mechanisms to gain access to the brain parenchyma. One such mechanism employed by tumor cells involves expression of a sialyltransferase, ST6GALNAC5 [47] (Fig. 2.1b(i)). ST6GALNAC5 expression was initially found enriched in “brain-tropic” human breast cancer lines. The authors subsequently tested whether or not this molecule was involved in mediating infiltration of the BBB by gain- and loss-of-function studies in mice. Overexpression of ST6GALNAC5 increased the ability of human breast cancer cells to traverse the BBB. Conversely, depletion of ST6GALNAC5 from “brain-tropic” breast tumor cells diminished extravasation and brain metastases back to basal levels [47]. Thus, unique but still poorly understood mechanisms are required to breach the BBB. Whether specific factors mediate extravasation within, e.g., the lung, bone marrow, and liver is currently undetermined.
Other Cells Involved in Extravasation
Thus far, we have focused on how tumor–EC interactions facilitate breakdown of the endothelial barrier and entry of circulating tumor cells into a tissue. However, other cell types within the circulatory microenvironment can also influence endothelial function and facilitate tumor cell extravasation. Platelets have long been known to play a role in tumor cell dissemination, and tumor cells activate platelets through multiple mechanisms [48–57]. Nevertheless, it was shown recently that activated platelets associated with tumor cells release adenosine triphosphate (ATP), which binds the P2Y2 receptor expressed on ECs. In response, EC–EC junctions relax, and tumor cells enter the parenchyma more easily [58]. There are a number of other cell types that doubtlessly contribute to vascular leakiness. Rather than enumerating every known interaction, below we present the unique concept that a tumor can affect leakiness from afar in order to facilitate metastasis .
Tumor-Derived Factors Influence Vascular Leakiness
Tumors are capable of aiding and abetting extravasation and survival of their disseminated seeds. The “pre-metastatic niche ” [59] refers to how the primary tumor can systemically influence the resident cells of a target organ, bias the factors they produce, and stimulate recruitment of pro-metastatic bone marrow-derived cells to the organ to prime it for the subsequent arrival of DTCs. This in turn facilitates the survival of DTCs and supports their expansion into micrometastatic foci. In particular, the recruitment and engraftment of VEGF1+ CD11b+ CD34+ bone marrow-derived hematopoetic progenitor cells in secondary sites precedes DTC arrival, during which time these cells remodel the local microenvironment in order to provide a more hospitable one for DTCs [59]. More recently, exosomes, cell-derived vesicles secreted both during physiological and pathological conditions, were discovered to dictate metastatic outcome due to their ability to effect a pre-metastatic niche [60]. Since they contain DNA, mRNA, miRNA, and proteins, exosomes facilitate intercellular communication and can result in activation of the recipient cell. Importantly, whether they are uptaken by ECs in a target organ or not, the first change exosomes effect at a secondary site is enhanced vascular permeability [61], which increases the ability of bone marrow-derived cells and tumor cells to enter and ultimately colonize the tissue.
Interchangeable Role of the Perivascular Microenvironment: Fostering Tumor Cell Dormancy and Malignancy
So far, our emphasis has been to understand how tumor cells and the endothelium interact to allow for specific steps (intra- and extravasation) of the metastatic cascade to occur. In this context, the endothelium is a physical barrier the tumor cell must circumnavigate to travel to a new site. Once there, it is becoming increasingly apparent that endothelial-derived cues dictate metastatic outcome. This should not necessarily come as a surprise, given the role the perivascular microenvironment has on organ development [62, 63], homeostasis [64–66], wound healing [66, 67], and in controlling stem cell quiescence and growth [68, 69]. Nevertheless, recent studies have shed light on how the endothelium and the microenvironment surrounding the endothelium, i.e., the PVN, dictate whether a DTC survives, enters a quiescent state known as cellular dormancy, and/or outgrows once it reaches a secondary site [70]. This opens up an exciting avenue for novel metastatic therapies [71], and further extends our view of the EC as an active player within the cellular microenvironment.
The Perivascular Microenvironment
The local microenvironment of a given cell refers to its anatomical location and the specific cell–cell interactions, ECM, and secreted factors that influence its phenotype. Signals that come directly from the microenvironment that result in a change within the cell are referred to as “non-cell autonomous” cues. Conversely, signaling events that can be initiated by the cell itself, without the influence of the local microenvironment, are referred to as “cell autonomous” cues.
A perivascular microenvironment (“peri,” around or near) simply refers to the immediate vicinity around vascular endothelium. The intricacies of tissue-specificity will mean that the perivascular microenvironment encountered by a tumor cell that has extravasated into the brain is different from that it would encounter in the liver, for example. Nevertheless, angiocrine (i.e., endothelial-derived [72]) factors and basement membrane proteins deposited by the endothelium and perivascular cells such as pericytes (that wrap around endothelial capillaries to provide structural support) are common components of the PVN. Only recently has it become apparent that the perivascular microenvironment plays diverse and paradoxical roles in determining cell fate depending on context. This is somewhat of a paradigm shift, given that it was only discovered in 2000 that endothelium actively directs tissue morphogenesis.
Endothelial-Derived Cues Instruct Organogenesis
The first insight that ECs functionally provide instructive cues, extending their role from what was thought to be limited to passive metabolic exchange, related to liver development [63]. Using a liver bud explant culture system, angioblasts were found to physically interact with hepatoblasts (hepatic endodermal cells that give rise to adult hepatic epithelium) prior to the detection of closed vascular structures. When ECs were either absent or inhibited in this system, the consequence was a dramatic defect in hepatic outgrowth [63]. This demonstrated for the first time the requirement for endothelial-derived signals in directing organogenesis. Similar studies in the pancreas confirmed signals from the aorta are necessary to instruct endocrine pancreatic cell differentiation during development [62]. Thus, a paradigm of activated/invasive ECs promoting growth and differentiating ECs fostering differentiation was born.
The Endothelium During Homeostasis and in Wound-Healing
Just as it is important to appreciate how endothelium functions to direct development, understanding how the endothelium functions both in adult homeostasis and wound-healing is necessary to fathom how it could operate in a metastatic setting. The liver provides an interesting case study.
Liver sinusoids are lined by liver sinusoidal ECs (LSECs) ; reviewed by [73]. LSECs are the only example of mammalian endothelium that combines lack of a diaphragm across their fenestrae with lack of a typical basement membrane. Furthermore, the fenestrations on LSECs are arranged into sieve plates. It is this unique phenotype that allows ideal contact and rapid macromolecule exchange between hepatocytes (liver epithelial cells) and blood while providing the necessary latticework for cellular structure and function [74, 75]. On the luminal side, LSECs are in direct contact with Kupffer cells (resident liver macrophages) that line the sinusoids [76, 77]. On the abluminal side is an extracellular space (“the space of Disse”) where hepatic stellate cells reside in direct contact with and support LSECs [78–80]. An important point to consider is that LSECs are in a prime position to directly contact Kupffer, hepatic stellate cells, and hepatocytes. Given this unique sinusoidal anatomy, hepatocytes have even been found to contact circulating T cells directly by extending cytoplasmic extensions through endothelial fenestrations and into the sinusoid [81].
LSECs and hepatic stellate cells—the equivalent of a liver pericyte—have a dynamic relationship. During liver homeostasis, quiescent LSECs prevent the activation of hepatic stellate cells (via nitric oxide (NO) secretion) and promote reversion of activated hepatic stellate cells to a quiescent phenotype [65]. Reciprocally, hepatocyte- and hepatic stellate cell-derived VEGF help maintain the physiologic phenotype of LSECs [64].
In response to epithelial damage, a liver wound healing response will ensue. This is a cellular reaction comprised of inflammatory, regenerative, and fibrogenic components that intrahepatic cell populations coordinate in an attempt to repair the liver and return to homeostasis (reviewed in detail by [82, 83]). Importantly, fibrogenesis is driven by activation of the hepatic stellate cell—which acquires a myofibroblast-like phenotype and begins secreting excessive ECM [84]. Liver injury is accompanied by activation of LSECs, a process called capillarization. Capillarization sees LSECs take on a more typical vascular phenotype, which is characterized by loss of fenestrae and abnormal/excessive deposition of basement membrane proteins into the PVN. Activated LSECs stop producing NO and can no longer support maintenance of the quiescent hepatic stellate cell phenotype [85]. Furthermore, activated LSECs increase production of fibronectin [86] and endothelin [87], which are drivers of hepatic stellate cell activation.
It is obvious then that within the liver microenvironment, LSECs are central players in maintenance of homeostasis, the wound healing response, and perpetuating fibrosis. In other organs, such as the lung and kidney, ECs and the PVN also direct fibrosis [88, 89]. For example, in the lung, repeated injury suppresses C-X-C chemokine receptor type 7 (CXCR7) expression on lung capillary ECs. This results in the recruitment of perivascular macrophages, which increases Jagged-1 ligand expression on ECs. Subsequently, Jagged-1 ligand binds to the Notch receptor on adjacent perivascular fibroblasts, which enhances fibrosis. Either CXCR7 agonists or knockdown of Jagged-1 in lung ECs skews the downstream cellular response from a fibrotic one to one more directed toward promoting repair [88]. Therefore, the endothelium lies at the crux of tissue repair and fibrosis in multiple organs.
A Perivascular Niche for Stem Cells, Across Tissues
How the PVN can simultaneously regulate the quiescence and growth of a cell is perhaps best illustrated by examining stem cell maintenance. The subventricular zone (SVZ) houses one of two adult neural stem cell (NSC) niches. In the mammalian brain, the subventricular zone [90]. Here, quiescent type B stem cells become activated type B stem cells, which give rise to transit amplifying type C progenitors that subsequently differentiate into type A neuroblasts. Both type B and C cells are found in direct contact with the vasculature; however they each have a unique interaction with the endothelium. Type B cells extend specialized end-feet projections that make stable contact with endothelium. On the other hand, type C cells make more transient endothelial contact, at smaller sites [69]. The nature of the contact with the endothelium dictates which signaling pathways are activated within type B/C cells, thus influencing whether NSCs remain quiescent (type B) or begin to proliferate/differentiate (type C) [69]. The brain endothelium expresses Jagged-1 and Ephrin B2, ligands that bind to Notch or Ephrin (Eph) receptors, respectively. Both type B and type C cells express these receptors, and importantly, ligand–receptor binding can only occur in the case of direct cell–cell contact. As type B cells are in tight contact with brain endothelium, Notch and Eph receptor signals are transduced simultaneously. This attenuates mitogen-activated protein kinase (MAPK) signaling, preventing growth factor-induced activation of cyclin D and cell cycle progression. It is this combination of Notch/Eph signal transduction that keeps type B cells quiescent. On the other hand, type C NSCs interact with the endothelium transiently, leading to insufficient engagement of Notch and Eph receptors. The result is growth factor-induced activation of the MAPK cascade, cyclin D accumulation, and cell cycle turn over [69]. This is a prime example of how the endothelium expresses specific factors that dictate both quiescence and cell cycle progression dependent upon the nature of the physical interactions between a cell and the endothelium.
Bone marrow stem cells (i.e., HSCs) are also regulated by the PVN. The great majority of HSCs localize to sinusoidal endothelium within bone marrow [91–93]. Stem cell factor (SCF) is a key maintenance factor of the HSC niche [94]. Deletion of SCF from various cellular compartments within bone marrow identified its cellular source [95]. Only deletion of SCF from the endothelial compartment or the Lepr+-perivascular stromal cell compartment led to HSC depletion [95]. This strategy of using cell-specific deletion of SCF allowed the authors to conclude unambiguously that HSCs reside within and are maintained by a PVN and that factors derived from this niche are responsible for maintaining HSCs.
However, EC phenotype is critical to this function. Specifically, angiocrine factors secreted from ECs signaling via an Akt-driven mechanism support self-renewal of long-term HSCs and expansion of hematopoietic stem and progenitor cells (HSPCs) [68]. Conversely, ECs transducing MAPK-driven signals drive HSPC differentiation and expansion [68]. This provides another striking example of how the EC is capable of dictating diverse cellular outcomes (quiescence versus proliferation) within a localized microenvironment, all depending on EC phenotype.
The preceding paragraphs describe examples of perivascular regulation of brain and hematopoietic stem cells. However, it should be noted that stem/progenitor cells in other tissues such as the liver, skin, skeletal muscle, the extramedullary HSC niche in the spleen [96], and also mesenchymal stem cells in the kidney, lung, heart, and liver occupy a PVN. The deduction of the endothelial/perivascular contribution to maintenance of these various stem cell niches is ongoing; nevertheless, it is clear that endothelial-derived signals are integral to controlling both quiescence and cellular growth under a variety of tissue-specific settings. How this paradigm applies to regulation of DTC quiescence and outgrowth is described below.
The Endothelium and Tumor Cell Dormancy
Once a DTC has extravasated into a distant organ, what are the scenarios that can subsequently play out? There are a number of possibilities (Fig. 2.2b). Firstly, if the tumor cell finds that the foreign microenvironment of the secondary organ it has invaded does not provide adequate survival cues, the tumor cell may die. Secondly, the DTC may begin slowly dividing, driven primarily by autonomous cues and emerge years down the road. Thirdly, the cell may encounter a favorable microenvironment—known as a “metastatic niche”—and initiate metastatic outgrowth. And finally, the cell may enter into a state known as cellular dormancy.
Not a great deal is known about how DTCs survive in foreign microenvironments. However, even here the PVN has been implicated, specifically signaling through heterotypic connexin gap junctions formed between ECs and DTCs that facilitate DTC survival [97]. If a DTC survives, what determines whether it falls asleep or whether it grows?
When discussing tumor dormancy, there are two distinctions to be made. There is a state known as “population dormancy”—in which proliferation and death are balanced within a micrometastatic nodule. This ultimately keeps the micrometastatic mass in a static growth state [98]. Escaping this state requires evasion of immune surveillance [99] and induction of the “angiogenic switch ” [100]. Thus, it was long assumed that the endothelium’s role in regulating metastatic colonization was a passive one; as long as new blood vessels were induced, outgrowth would occur.
However, a second and possibly more frequent type of tumor dormancy also exists that is not restricted by nutrient availability. This state, known as “cellular dormancy,” describes a single tumor cell or small cluster of tumor cells that enter G0 of the cell cycle and are mitotically arrested in secondary sites for an unspecified period [101]. The remaining discussion will deal specifically with this state of dormancy. Cellular dormancy is an amazing phenomenon in that of the ~30% [102] of breast cancer patients who will develop distant metastases, 20% of these clinically disease-free cases will relapse 7–25 years after adjuvant therapy [103]. How do these single cells/small clusters of cells persist for such a long period of time within a tissue? Further, what cues subsequently drive them to reemerge?
The first microenvironment a DTC will encounter upon entering into a new tissue is a perivascular one. Perhaps it is no surprise then that dormant disseminated breast tumor cells reside within a PVN in vivo. Dormant DTCs were found in a PVN in all target organs that breast cancer commonly metastasizes including the bone marrow, lung, brain [70], lymph nodes, and liver (Lim et al.; Grzelak et al., unpublished work). Having deduced that the breast cancer dormant niche was a perivascular locale, Ghajar, Bissell, and colleagues commenced to interrogate the perivascular cues that sustain breast cancer cell quiescence. Using a proteomics-based approach, the authors were able to narrow down a candidate list of ECM-derived “dormancy” factors expressed highly within the PVN. Intriguingly, thrombospondin-1 (TSP-1), long known as an anti-angiogenic factor [104], was identified [70]. TSP-1 was expressed both in culture and in vivo along the length of vessel “stalks”—stable regions along the endothelial vessel not undergoing endothelial remodeling. Gain- and loss-of-function studies demonstrated that TSP-1 functioned to prevent breast cancer cell outgrowth. Therefore, TSP-1 is at least one PVN factor, secreted from ECs, that has an apparent role in controlling breast cancer tumor cell dormancy in the lung and bone marrow. Another EC-derived factor shown to suppress tumor cell growth is perlecan, the major heparin sulfate proteoglycan expressed by ECs. Knockdown of perlecan in ECs resulted in ECs that were no longer able to suppress the invasiveness of breast or lung cancer cells. Further, mice injected with lung cancer cells pretreated with EC-conditioned media, rather than EC-conditioned media from perlecan-silenced ECs, had reduced metastatic burden [105]. This indicates perlecan is another PVN-derived factor that may also function to maintain tumor cell dormancy.
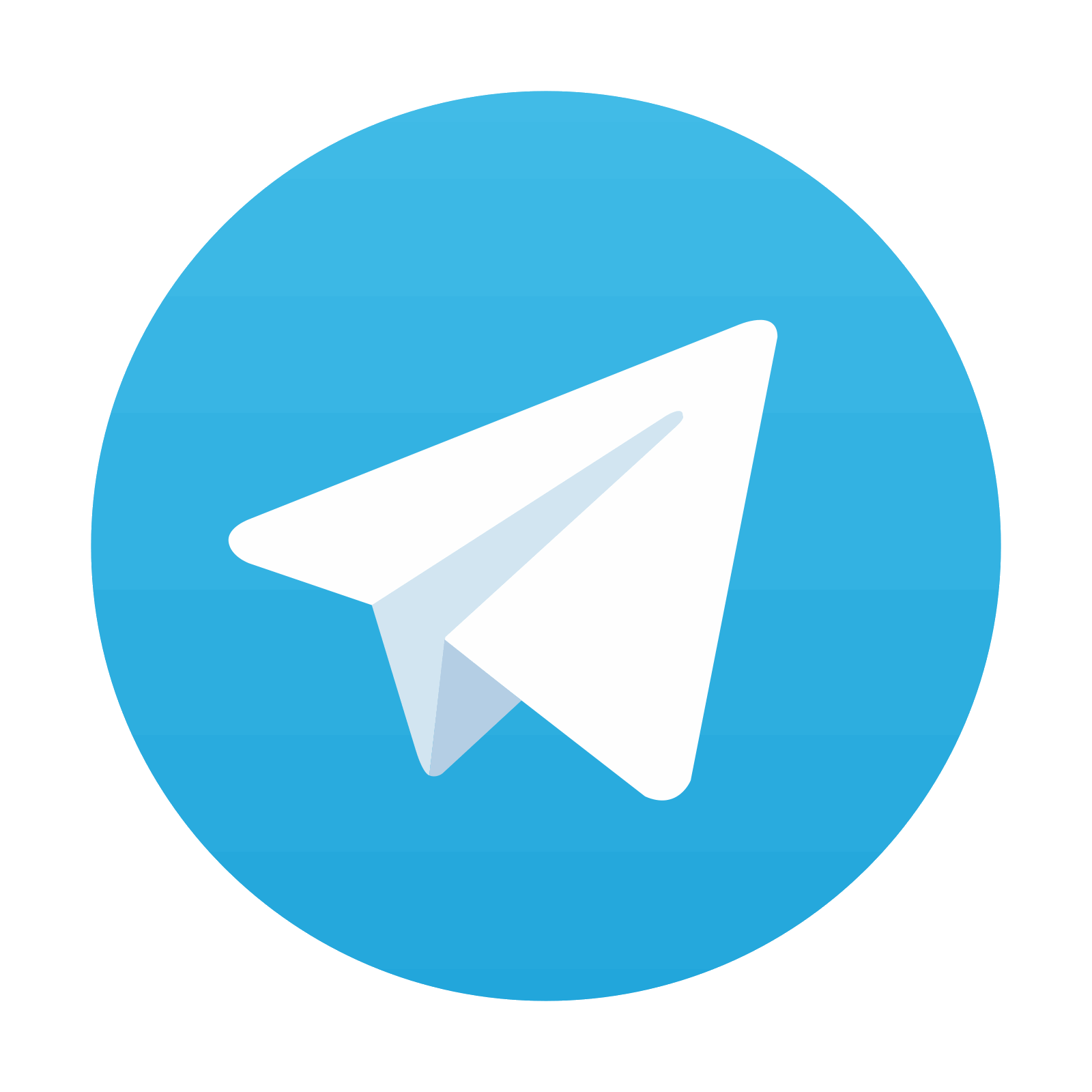
Stay updated, free articles. Join our Telegram channel
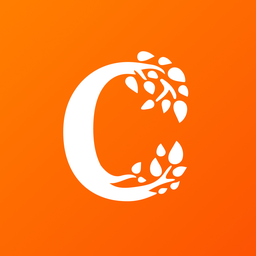
Full access? Get Clinical Tree
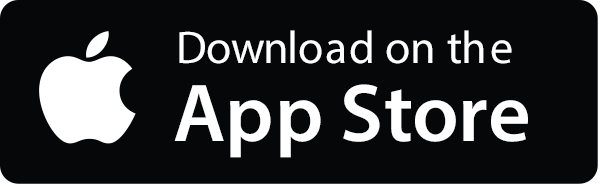
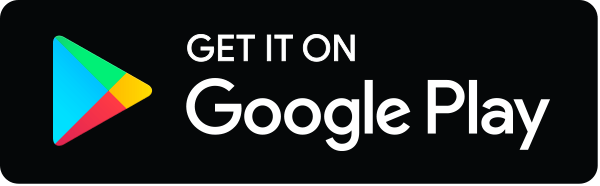