Chapter 6
Molecular Endospectroscopic Approaches
6.1 Introduction
Light can interact with cells and tissues in a number of ways, including elastic and inelastic scattering, reflection, and absorption, which can lead to fluorescence and phosphorescence. These interactions can be used to measure changes in the morphology and molecular composition of tissues. Optical biopsy systems have the potential to be used as an adjunct to current investigative techniques to improve the targeting of blind biopsy. More advanced molecular endospectroscopy techniques are likely to provide real-time, highly sensitive, and specific measurements of the pathological state of the tissue. They have the potential to identify markers associated with malignant changes and could be used as a diagnostic tool for the early detection of precancerous and cancerous lesions in vivo. The clinical need for an objective, noninvasive, real-time probe for the accurate and repeatable measurement of pathological state of the tissue is overwhelming. There are numerous clinical applications where endospectroscopy could have a significant impact in advancing early diagnosis, biopsy targeting, and disease monitoring. This is particularly important for cancers lining the surface of organs, where it may be possible to gain access via fiberoptic or other wave-guiding technology.
This chapter discusses some of the recent advances in the field and compares some of the approaches taken to achieve this goal.
6.1.1 Clinical Need
According to the GLOBOCAN database, approximately 12.7 million new cancers were diagnosed worldwide in 2008 [1]. During the same year, 7.6 million deaths globally could be attributed to cancer, representing 13% of all deaths [1]. Advances in cancer diagnostics play a pivotal role in increasing early detection of cancer and improving the chances of successful treatment. Ongoing research has demonstrated that many cancers develop through pre-malignant stages. Eradication of these abnormal cells prior to systemic invasion will prevent the development of invasive cancer. The clinical need for techniques that can accurately identify these pre-malignant biochemical changes is considerable.
Diagnostic pathways are complex and may involve many diagnostic tests such as planar X-ray, computed tomography, ultrasound, and endoscopy, but currently histopathology remains the “gold standard” investigation required to provide a definitive diagnosis. This method is invasive, requiring the removal of tissue, often unnecessarily, and sometimes requires the detection of invisible lesions using random targeting protocols. The processes of tissue fixation, sectioning, and staining that follow can be costly and slow. Histological analysis is highly subjective and is associated with considerable inter-observer disagreement [2], as was outlined in Chapter 1. This is particularly the case for early lesions, where minimally invasive treatments are likely to be most effective.
Cancers developing in epithelial tissues account for 85% of cancers [3]. Epithelial tissues line most organs and are exposed to numerous varied carcinogens throughout life. On the flip side, as epithelial tissues line hollow organs, many are accessible using endoscopic technologies; furthermore, many malignancies are known to develop over long timescales and transition through pre-malignant stages. These are prime candidates for early diagnosis using novel endospectroscopy, particularly if populations at risk of specific malignancies can be identified.
Three organs where there is significant incidence of epithelial cancer and where endospectroscopy can have a significant impact are discussed further in the following sections.
6.1.1.1 Esophagus
The incidence of esophageal adenocarcinoma has increased by 500% over the past 40 years, making it the seventh most prevalent cancer in the United States and the sixth most common cause of cancer-related mortality [4]. In 2010 in the United Kingdom, there were 8477 cases of esophageal cancer diagnosed, and 7610 individuals died from the disease [5].
Patients typically present with advanced disease, which precludes curative resectional surgery, and 5 year survival rates have therefore remained poor (8–15%) [4, 6]. Identification of patients at an early premalignant stage could enable curative endoscopic or surgical treatment, thus preventing progression to malignancy.
Esophageal adenocarcinoma is known to develop through a sequential series of cellular changes in the esophageal mucosa (the innermost lining of the esophagus), which may occur in response to chronic acid reflux. Initially, there is a metaplastic change from a normal squamous epithelium to a columnar cell epithelium known as Barrett’s esophagus, which confers a 100-fold increased risk of developing cancer [7].
Endoscopic identification of precancerous dysplasia within Barrett’s esophagus could enable early minimally invasive therapeutic intervention, which could prevent malignant progression. However, detection of dysplasia using routine white-light endoscopy (WLE) is notoriously difficult and subjective even for experienced clinicians. This is particularly the case when discriminating between the clinically important high-grade dysplasia (HGD) and low-grade dysplasia (LGD) [8, 2].
There are problems with targeting the locations of early cellular lesions, which may be important if diagnosis is to be made prior to invasive cancer developing. Figure 6.1 outlines the sampling problem within an average region of Barrett’s esophagus.

Figure 6.1 Approximate biopsy area versus the 4 cm mean region of Barrett’s esophagus, requiring random biopsy to detect invisible dysplastic lesions. If the esophagus diameter is 2.5 cm and the biopsies are around 1 mm2, then the relative sampled area is around 0.5%, or 99.5% of the region remains unsampled. The bottom right image shows the number of biopsy samples taken in a regular upper endoscopy procedure.
Once the biopsies have been sampled, a further barrier to accurate diagnosis is histopathology, which is unreliable and can lead to inter- and intra-observer agreement of around 50% for key early disease conditions [8]. Histological diagnosis of dysplasia is extremely subjective, and wide ranges of inter-observer variation (using Kappa statistics where 0 is chance agreement and 1 is perfect agreement) with K-values of 0.2–0.6 have been reported, potentially resulting in unnecessary interventions or omission of important clinical surveillance [9].
6.1.1.2 Colon
Colon cancer is the fourth most common cancer in the United Kingdom, making up 13% of all new cases in 2010, with 40 695 new cases diagnosed. Five-year survival in the United Kingdom is currently 55%. Approximately 60% of colorectal carcinomas arise in the region between the descending colon and rectum/anus [10]. The remaining ones are distributed roughly equally throughout the rest of the colon. During colonoscopy, polypoid lesions are commonly found. Adenomatous polyps are known to be pre-malignant and should prompt immediate removal. However, these dysplastic lesions may be difficult to distinguish from benign hyperplastic polyps, which do not have malignant potential. In addition, distinction between the two major forms of inflammatory bowel disease (ulcerative colitis (UC) and Crohn’s) is frequently impossible during colonoscopy. UC can predispose the sufferer to the development of microscopic dysplasia within the areas of inflammation. However, this is not visible using conventional colonoscopy, and therefore common clinical strategies involve taking multiple random biopsies in an effort to identify these early dysplastic changes.
6.1.1.3 Bladder
The incidence of bladder cancer in the United Kingdom is 8% in men and 3% in women [11]. It is the fourth most common cancer in men and the eighth in women. Each year, there are over 10 000 cases diagnosed in the United Kingdom with 4907 deaths (56% of patients survive for 5 years post diagnosis). There has been a reduction in the age-standardized incidence since the 1980s [12]; it is thought that the reason for this is the reduction in smoking and the banning of aromatic amines in the 1980s. Both of these are known risk factors for the development of bladder cancer.
Ninety percent of bladder cancers are transitional cell carcinomas (TCCs) of the bladder, and the majority of these are superficial. The accessibility of the bladder to fiberoptic-based cystoscopy has led a number of groups worldwide to explore the use of optical techniques. These are outlined later in this chapter.
6.2 Endoscopic Imaging Techniques: Sampling Tissue Morphology/Architecture
All three organs, discussed above, require novel tools able to provide minimally invasive, accurate, reproducible, rapid, nondestructive, and cost-effective detection and diagnosis of early malignancies. This next section will explore the wide-field imaging methods available for probing the cellular morphology and tissue architecture (more detail to be found in Chapter 2). Many of the endoscopic imaging tools presented here have been trialled in the esophagus, bladder, and colon; however, there remains a significant clinical need for a diagnostic tool that could enable objective diagnosis of dysplasia in vivo [13].
6.2.1 High-Resolution Endoscopy (HRE)
Modern high-resolution endoscopes (HREs), which generate up to and greater than one million pixel images (compared to the 300 000 pixel images of traditional scopes), have been shown to have a higher sensitivity than standard WLE for the detection of early Barrett’s neoplasia, provided they are used by expert endoscopists [14, 15]. To utilize the benefits of these endoscopes, they should be used in conjunction with high-definition television monitors to further enhance the projected image quality and prevent loss of resolution when larger images are required.
Kara et al. [15] showed that, even in the hands of experts, targeted biopsy using HRE was only capable of detecting 79% of dysplasia, and differentiation between HGD and LGD for the purposes of targeted treatment was difficult. Clearly, HRE should replace standard WLE where possible; although to significantly improve endoscopic diagnosis of dysplasia, HRE may be best utilized in conjunction with additional complementary endoscopic tools for a more advanced diagnosis.
6.2.2 Confocal Microscopy (CM)
Confocal microscopy (CM) magnifies the mucosa 1000-fold, enabling real-time visualization of cellular structures. CM has shown considerable potential in Barrett’s esophagus, with reported accuracy of up to 97.4% for the detection of dysplasia [16]. However, because of considerable inter-user variation, these results have not been universally matched [17]. The technique has also been criticized for being both time consuming and expensive. Furthermore, it requires considerable training to interpret, and relies upon the use of exogenous contrast to demonstrate the irregular neovascularization that is characteristic of neoplastic tissue. Further exploration of the relative potential of this technique is required before it can reach widespread use.
6.2.3 Chromoendoscopy (CE)
Chromoendoscopy (CE) involves exogenous administration of stains to the esophageal mucosa in order to improve tissue characterization during endoscopy. Absorptive stains (such as methylene blue) cross epithelial membranes selectively, whereas contrast stains (such as indigo carmine) permeate into mucosal crevices highlighting surface topography and mucosal irregularities [18].
Studies utilizing CE in Barrett’s esophagus have had mixed results and have highlighted problems such as difficulties in uniformly coating the esophageal mucosa with the stain, and the excessive times necessary for stain-spraying [19, 20, 9]. The technique has not been shown to consistently outperform HRE in the detection of early neoplastic lesions [21].
CE is relatively widely available and does not require any particular equipment except for a spray catheter, which is simple to use and cheap to purchase. However, CE has been shown to be both labor intensive and operator dependent and is therefore unlikely to become widely utilized.
6.2.4 Narrow-Band Imaging (NBI)
Narrow-band imaging (NBI) illuminates the mucosa with white light and selectively measures the blue and green detector channels (in a three-channel camera) in order to enhance the detail from the mucosal surface, by relying on the principle that longer wavelengths of light penetrate deeper into tissue than shorter wavelengths. Narrow-band blue light displays the superficial capillary networks, while green light displays the subepithelial vessels, and a combination of the two images produces an extremely high-definition image of the mucosal surface allowing visualization of subtle mucosal irregularities and alterations in vascular patterns consistent with dysplasia and intramucosal carcinoma (IMC) [22].
NBI is a widely available technique that avoids the need for staining or intravenous contrast agents. It has shown promise in the detection of dysplastic lesions when in the hands of experienced users [22, 23]. A recent review of NBI with magnification demonstrated high accuracy for the diagnosis of HGD in Barrett’s esophagus based on the recognition of irregular mucosal pit patterns and/or irregular microvascularization [24]. However, NBI is time consuming and results have been mixed because of high levels of inter-observer variability [22, 21]. Overall, data on the accuracy of NBI in Barrett’s esophagus are inconclusive and results of multicenter randomized controlled trials are awaited.
6.2.5 Optical Coherence Tomography (OCT)
Optical coherence tomography (OCT) is analogous to ultrasound imaging but can produce higher resolution images (≈1 μm) as it relies on backscattering of near-infrared (NIR) light (which has much shorter wavelengths than the sound waves used in ultrasound) to generate cross-sectional images of epithelial and sub-epithelial tissues.
OCT is performed using probes passed through the instrument channel of endoscopes. It does not require the use of exogenous contrast agents and, unlike with ultrasonography, tissue contact is not required.
Several studies have assessed the role of OCT in the detection of dysplasia. In a study of 55 patients with Barrett’s esophagus, OCT was shown to delineate between HGD and adenocarcinoma with a sensitivity of 83% and a specificity of 75% [25]. Another study of 33 patients demonstrated a diagnostic accuracy of 78% for the identification of dysplastic Barrett’s esophagus; however, considerable user discrepancy (56–98%) was demonstrated [26].
Further clinical evaluation is required to assess the diagnostic performance of OCT in the esophagus. The key outcomes of the various studies highlight the requirement for subjective image interpretation, which, coupled with the low contrast of most OCT images of epithelial tissues, becomes a very difficult task for early disease detection. Figure 6.2 shows typical images from epithelial tissues. In the future, the technique may have a greater role in the staging of early esophageal tumors rather than in the detection of dysplasia; however, the considerable time required to acquire and interpret the images may become a significant limiting factor.

Figure 6.2 Representative OCT images of porcine samples. (a) Squamous lining of the esophagus, (b) tongue, (c) tracheae, and (d) bronchiole. Each image covers 3 mm in width [27].
A number of recent studies have attempted to overcome the problem of OCT image interpretation for diagnosis, by taking automated approaches utilizing the fact that the OCT images consist of profiles of light scattering, whereby the slope of the light intensity with depth or attenuation appears to correlate closely with disease [27–30]. In addition, work to optimize the wavelength to maximize contrast and produce an acceptable sampling depth trade-off shows some value [31].
6.2.6 Novel Imaging Techniques: Summary
Currently, none of these advanced endoscopic imaging techniques has yet been widely adopted into clinical practice. This reflects a variety of limitations, including insufficient sensitivity or specificity, inter-observer variability, cost, and technical difficulties. Furthermore, all of them have attempted to utilize measures of tissue and cell architecture and morphology, rather than to probe molecular constituents and tissue function accessible with spectroscopic approaches. There is a continuing need for a novel imaging technique that could enable objective assessment of epithelial tissues in hollow organs in order to minimize missed disease and aid targeted endomucosal resection (ER), photodynamic therapy, radio frequency ablation, or other conservative treatments for early disease.
The advent and now widespread use of ER in the esophagus has meant that accurate targeting of focal HGD/IMC has become essential. A study by Vieth et al. [13] showed that a complete resection was achieved in just 26.8% (87/326) of patients at the first attempt because of inadequate endoscopic assessment of lesion margins. This improved to 74.8% (244/326) following repeat endoscopy. The study concluded that failure to achieve complete resections at the initial endoscopy would introduce an unnecessary time delay and may increase complication rates, and the development of improved endoscopic imaging techniques was strongly advocated.
6.3 Molecular Endospectroscopic Techniques: Probing Native Molecular Signals
The previous section illustrated the current position with regard to advanced endoscopic diagnosis in epithelial malignancies. It is hoped that molecular endospectroscopic techniques may overcome many of the pitfalls associated with these alternative techniques. These are outlined in the following section.
6.3.1 Autofluorescence Imaging (AFI)
Autofluorescence imaging (AFI) detects fluorescence from naturally occurring fluorophores following excitation of tissue using light of short wavelengths. This is usually in the blue range (425–455 nm), and longer wavelength photons subsequently emitted are measured. This enables the technique to measure the variation in the type and concentration of fluorophores with diseased conditions, thus enabling some differentiation between normal, metaplastic, and neoplastic tissues. The main signals measured appear to originate from collagens, flavins, and nicotinamide adenine dinucleotide hydrogenase (NADH). Several studies have suggested that AFI is sensitive for the detection of HGD in Barrett’s esophagus although the technique appears limited by low specificity [32–34]. Curvers et al. [35] demonstrated an increased detection rate of HGD/IMC using AFI compared to WLE alone (53% vs 90%), but this came at the expense of a high false positive rate of 81%.
6.3.2 Fluorescence Lifetime Imaging (FLIM)
Fluorescence lifetime imaging (FLIM) has some advantages over conventional imaging of autofluorescence signals. It relies upon the simultaneous measurement of the temporal decay in fluorescence intensity following laser excitation at every spatial point in a sample, and imaging intrinsic tissue autofluorescence from biomolecules such as NADH, elastin, and collagen. FLIM can provide functional images by mapping the spatial distribution of fluorescence lifetimes. FLIM can be performed either in the frequency domain, in which a high-frequency modulated laser beam excites the sample and the fluorescence lifetime is determined from the demodulation and phase shift of the fluorescence signal, or in the time domain, in which the fluorescence decay is directly measured after pulsed laser excitation. With either approach, functional information may be derived from the fluorescence lifetime by means of its dependence on the radiative decay rate and the nonradiative decay rate of the fluorophore. As a consequence, the fluorescence lifetime is not only sensitive to the type of fluorophore but also depends on its environment. A number of studies have now shown that FLIM has the ability to provide intrinsic contrast between different tissue states [36], including between healthy and diseased tissues [37, 38].
Extensive development of this technology to endoscopic systems has led to the capability of measuring tissue-discriminating fluorescence lifetimes through endoscopes. However, initial work demonstrated the time requirements of greater than 5 min per image [39], which has been improved subsequently to 29 wide-field fluorescence-lifetime images per second using 355 nm excitation [40], thus making in vivo implementation more feasible. Weinigel et al. [41] were able to perform in vivo measurements using FLIM endoscopy with the medically certified multiphoton tomograph MPTflex® in combination with a computer-controlled motorized scan head and a gradient index (GRIN)-lens endoscope. Cheng et al. [42] have also developed a compact wide-field time-gated FLIM flexible endoscope capable of continuous lifetime imaging of up to three fluorescence emission bands simultaneously. They plan to undertake future in vivo measurements. There is clearly some potential with FLIM for in vivo molecular endospectroscopic imaging in real time. Numerous studies are planned, which are required to provide evidence of accurate identification and discrimination of early malignancies.
6.3.3 Elastic Scattering Spectroscopy (ESS)
Elastic scattering spectroscopy (ESS) relies upon the white-light illumination of tissues and cells to provide a backscattered signal. This signal correlates with both the size and shape of the cell nuclei, the degree of cellular crowding, and chromophore absorption, such as hemoglobin. Nonabsorbed photons are backscattered to the surface through various molecular interactions. The photons with wavelengths most similar in size to the most abundant scatterers in the tissues probed will be the most represented in the measured signal at the surface. This phenomenon is described as Mie scattering. Clearly, where chromophores able to absorb scattered light are present, they will reduce the intensity of specific wavelengths.
This technology can be cheaply implemented, as it measures a highly abundant signal so can utilize low-cost optical components. Fiberoptic probes can be passed through the instrument port of an endoscope to generate morphological information about the nature of the region of interest from the epithelial lining of the organ. Spectral signals can be acquired in short acquisition times approaching “real-time” imaging of the mucosa and submucosa with varying signals depending on the disease-related size and shape of the cell nuclei and the degree of cellular crowding.
Dysplastic and malignant tissues have been shown to have a characteristic ESS signature; however, as a result of the signal interference from deeper structures, the accuracy of ESS appears limited to around 85% [43]. Lovat et al. measured spectra from 181 tissue sites from 81 patients, which were correlated with consensus histopathology. ESS identified HGD with a sensitivity of 92% and a specificity of 60% and was able to differentiate these sites from inflammation with a sensitivity and specificity of 79% [44]. This current level of accuracy was deemed insufficient to support clinical uptake of ESS unless it can be improved through refinement of the technology or the use of a concomitant imaging modality.
Recently, Qiu et al. [45] described a novel endoscopic polarized scanning spectroscopy (EPSS) system capable of performing rapid optical scanning of the esophageal surface in near real time. The technique records elastically scattered light and filters signal from deeper tissue, enabling epithelial interrogation. Five patients with Barrett’s esophagus underwent EPSS. Ninety-five biopsies were taken for comparison with spectral data which, compared to a histological gold standard, demonstrated a sensitivity of 92% and a specificity of 96% (all pathologies). Further ongoing development and more extensive testing of this technology is necessary before sound conclusions on its potential for widespread clinical use can be drawn.
6.3.4 Raman Spectroscopy (RS)
Raman spectroscopy (RS) is beginning to gain recognition as a potential tool for endospectroscopy because of the molecular specificity of the technique. Recent advances in RS have promoted it to a level at which in vivo trials are beginning to emerge. When used in combination with powerful multivariate algorithms, which analyze the detailed molecular fingerprints obtained, the technique can potentially provide automated, objective, and reproducible classification of pathology in clinically relevant time frames.
RS enables the elucidation of a tissue’s biochemical fingerprint by measuring the molecular-specific inelastic scattering of light. Primarily, RS requires the illumination of a tissue sample with a monochromatic light source, such as a laser, and subsequent collection and analysis of the scattered light for intensity and wavelength.
Photons in the incident laser light undergo inelastic collisions with molecules, causing an exchange of energy and therefore a change in frequency. The frequency change, known as the Raman shift, is specific to the species of molecule causing the scattering and can be defined as the difference between incoming and scattered photon frequencies. Larger shifts indicate that more energy is required to bring about a particular vibrational motion.
The frequency or energy shift of the inelastic photons are independent of the wavelength of excitation, which means that the spectral peaks are measured at the same place no matter which wavelength is used for excitation, provided the spectrum is plotted against an ordinate which is proportional to energy. In the case of vibrational spectra (infrared absorption and Raman), this is usually plotted as wavenumbers (cm−1), which is the reciprocal of the wavelength of the light. The intensity of the inelastically scattered light is directly proportional to the concentration of the sum of molecular constituents giving rise to that peak. Thus the Raman spectrum is a direct function of the molecular composition of the interrogated volume within the tissue, giving us a complex molecular fingerprint (Figure 6.3).

Figure 6.3 Raman spectrum of esophageal tissue measured using 830 nm excitation. Some characteristic biochemical peaks have been labeled. Variations in peak height and position can be detected in tissue spectra and have been shown to indicate biochemical progression toward malignancy.
Fortunately, most biological molecules are Raman active, each with their own specific fingerprint. As a result, RS is highly sensitive to subtle biochemical and molecular changes, which is vital in the differentiation of tissue samples. It is this property that makes it potentially a very powerful diagnostic tool. Figure 6.4 shows some representative Raman spectra from various important biomolecules.

Figure 6.4 Mean spectra of the constituents used in the concentration fit. They include the main contributors to the Raman signal found in the tissues studied [46].
6.3.4.1 Introduction to Diagnostic Raman Spectroscopy Techniques
Over the past decade, progress in the throughput and sensitivity of RS instrumentation has made it possible to acquire high-quality spectral data from biological tissue in short timescales. As outlined in Chapter 3, these benefits have led to numerous developments for potential clinical tools [47].
Some ex vivo work on bulk tissue has been carried out, but most ex vivo studies have been performed on tissue sections. There has been a trend toward imaging/mapping approaches and the so-called “histopathology tools” because they mimic the morphological appearance of current histopathology methods but include additional biochemically specific information, without recourse to stains. RS has demonstrated its potential in aiding histopathologists in the identification and objective classification of subtle biochemical changes related to carcinogenesis [48–50].
As discussed previously, Raman mapping has potential as a “histology tool,” as it can provide prompt objective assessment of biopsied tissue samples without the need for tissue fixation or staining. Although this chapter is not focused on mapping applications of Raman as a histology tool, it is useful to note that these Raman mapping studies provide information relating to the biochemical constituents within tissue pathologies, which can be used to support Raman probe applications and influence and validate probe design. Shetty et al. demonstrated that RS was capable of detecting subtle alterations in the concentrations of a variety of biomolecules within esophageal tissues at different stages of progression toward malignancy [51]. Following least-squares fit analysis of pure spectra to the spectral datasets, higher concentrations of DNA, oleic acid, collagen I, and actin were demonstrated in dysplasia, with a predominance of glycogen identified in normal squamous tissue. Stone et al. [46] explored the use of NIR RS to provide a picture of the gross biochemical changes associated with the process of carcinogenesis in the bladder. Frozen biopsy specimens covering five pathology groups were measured and compared with spectra from purchased biochemical constituents. The biochemical basis for these pathologies was estimated using ordinary least-squares fitting. The results are shown in Figure 6.5. They provide some insight into the gross biochemical changes in the disease-specific compositional changes: advancing malignancy appeared to lead to increases in lipids, reductions in collagens, and increases in DNA to cytoplasmic ratios in these cases studied. De Jong et al. applied RS to discriminate between nontumor and tumor bladder tissues from 15 patients. Biochemical differences between tumor and nontumor areas were analyzed by fitting spectra of pure compounds to the tissue spectra. Nontumor spectra showed higher collagen content, while tumor spectra were characterized by higher lipid, nucleic acid, protein, and glycogen content [53].

Figure 6.5 Bar graph showing the relative concentration of each constituent for each bladder pathology, calculated using a least-squares fit of pure spectra from spectroscopic grade chemicals, normalized to unity. The pathology codes are marker on the x-axis and further outlined in the text [46].
The utilization of RS in vivo offers the potential for “optical biopsy” with important clinical benefits. Excisional tissue biopsy carries the risks of bleeding and visceral perforation, as well as removal of normal tissue unnecessarily. RS would obviate the need for this, thus reducing trauma to the patient, clinician workload, and secondary repeat procedures. It could also decrease the rate of re-excisions of areas of dysplasia or malignancy by accurately defining resection margins [54]. RS is suitable for use with fiberoptic probes, making it potentially ideal as a medical diagnostic tool for assessment of hollow organs [8, 53–56]. In addition, there are probe-based ex vivo applications emerging, such as the intraoperative assessment of lymph nodes, for the rapid identification of metastasis [57–60].
“Deep Raman” spectroscopy is a further extension of the technique that is being developed for application in noninvasive diagnosis of solid organs that can be achieved from outside of the body. At this stage of development, it can only be applied to specific applications where the Raman signature of interest is strong and distinct from the signals of the surrounding tissues, particularly when used at depths of many millimeters to centimeters, as there is a trade-off with the intensity of the signature obtained from depth [61–63].
RS can provide rapid, reproducible, and nondestructive measurement of disease-specific tissue composition, which when combined with powerful mathematical algorithms allows objective classification. Numerous spectral classification models are under development for both optical biopsy (probe) applications [56] and histology tool (mapping) applications [8, 48, 50]. Initially, early studies focused on differentiating normal tissue and advanced cancers. However, with developing technology and complex analytical methods, there has been a move toward diagnosis of neoplastic change at much earlier stages.
6.3.4.2 In Vivo Raman Endospectroscopy
Raman diagnostics in three key organs are discussed in this chapter to illustrate recent areas of development with a focus on real-time molecular endospectroscopy. This section will summarize the most up-to-date advances in RS, which demonstrate its potential as an endoscopic tool in the detection and management of cancer.
The Raman cross section is particularly low for tissue constituents, and the signals are relatively weak compared to other optical approaches. This means that, although the spectra are rich in molecular-specific information, they are limited by the time that is required to measure sufficient signals with safe power densities of laser light. Therefore, measurement of the native Raman signals, particularly down optical fibers, is generally restricted to spectral point measurements rather than imaging. Various approaches have been taken to develop probes of varying levels of complexity to sample different tissue volumes and to reject unwanted signals from reaching the spectrometer.
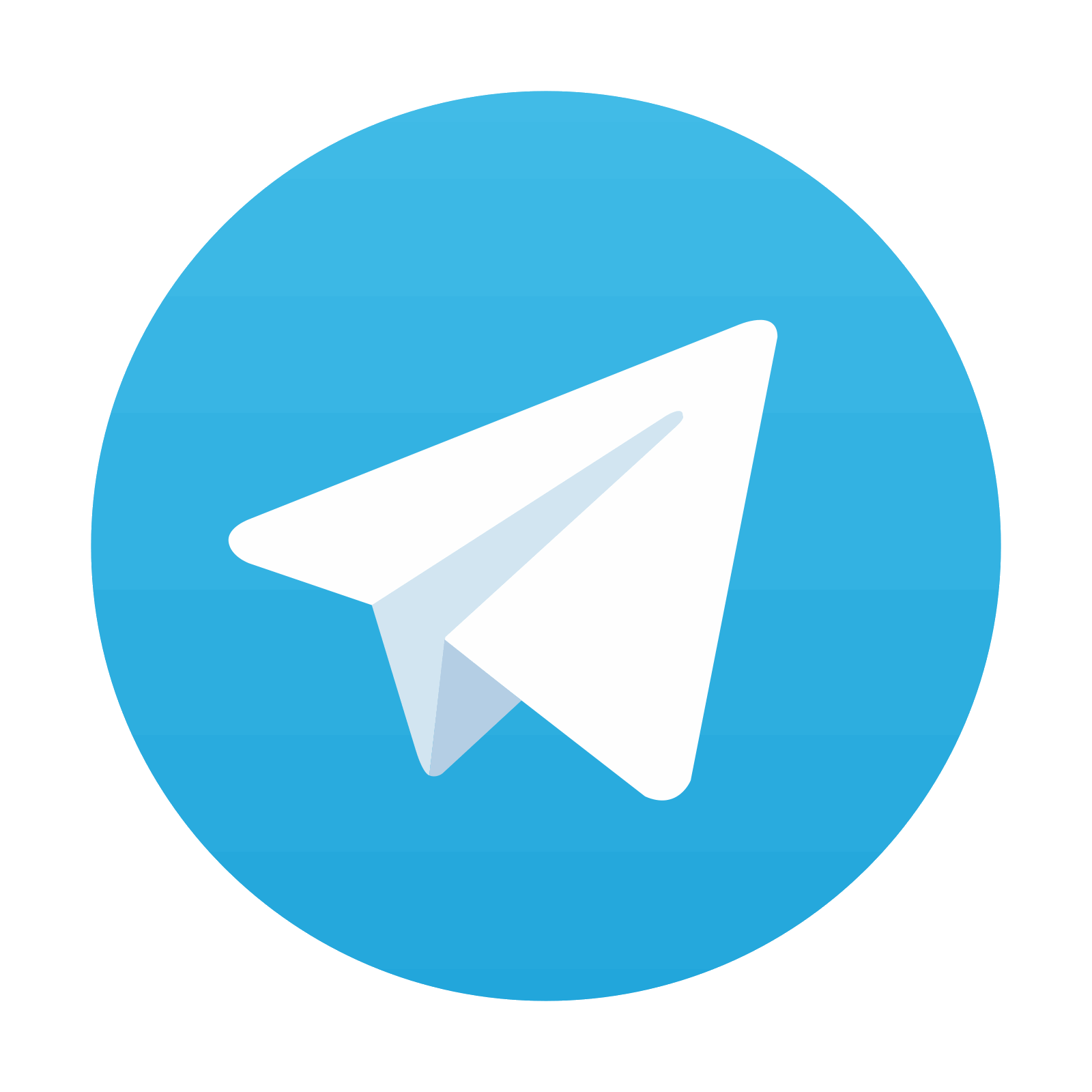
Stay updated, free articles. Join our Telegram channel
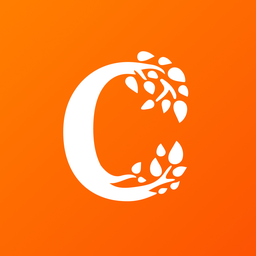
Full access? Get Clinical Tree
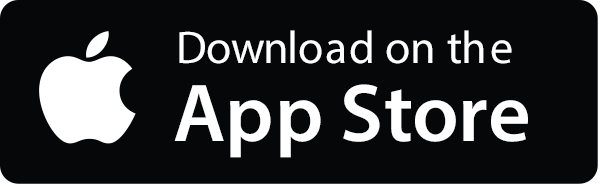
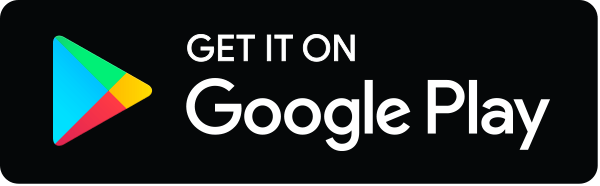